The interaction which occurs when a hydrogen atom, covalently bonded to an electronegative atom (as in AH), interacts with another atom to form the aggregate A
H
Y. The shortest and strongest bond is indicated as A
H, while the secondary and weaker interaction is written as H
Y. Thus A
H is a proton donor, while (Y) is a proton acceptor which often contains lone pair electrons and can act as a base. The strongest hydrogen bonds are formed between the most electronegative (A) atoms such as fluorine, nitrogen, and oxygen which interact with (Y) atoms having electronegativity greater than that of hydrogen (C, N, O, S, Se, F, Cl, Br, I). The weakest of hydrogen bonds are formed by acidic protons of C
H groups, as in chloroform and acetylene, and by olefinic and aromatic π-electrons acting as (Y).
Bond energies
The majority of hydrogen bonds have energies in the range 4–6 kcal/mole (17– 25 kilojoules/mole) and involve those between OH functional groups (as in water, alcohols, or acids) or N
H groups (as in amides or amines) and oxygen atoms (as in water, alcohols, carbonyls, or esters). The strongest hydrogen bond known is that found in the hydrogen difluoride ion, (F
H
F)-, which has been variously estimated at 37–55 kcal/mole (155–230 kJ/mole). Therefore, the average hydrogen bond is of much lower energy than a normal chemical bond (>100 kcal/mole or 418 kJ/mole). Although hydrogen bonding gives rise to a specific interaction between atoms, resulting in a complex with characteristic A
H
Y distances and angles, especially in the solid state, it is difficult to establish a lower limit for the H-bond enthalpy because experimental methods of detection are becoming increasingly more sensitive and accurate.
The weaker the hydrogen bond, the shorter the lifetime of the complex it forms. The detection of weak hydrogen bonds often amounts to measuring shorter and shorter lifetimes of rapidly associating and dissociating species in equilibrium. This is a difficult problem because proton transfer along hydrogen bonds belongs to the fastest known chemical reactions, and in most experimental studies only mean values and “average” structures are determined.
An important aspect of weak hydrogen bond formation is that the different molecular aggregates which do form can be easily and reversibly transformed. Thus the small energy changes resulting in the rapid making and breaking of hydrogen bonds in biological systems are of great importance; for example, hydrogen bonding determines the configuration of the famous α-helix, and the structures of most proteins, thereby serving an important function in determining the nature of all living things. See also: Deoxyribonucleic acid (DNA); Protein
Spectroscopy
Even though slight energy changes are usually involved in hydrogen bond formation, the aggregate once formed changes almost every measureable physical property of the original species. When investigating hydrogen bonding, the most frequently used techniques are infrared and nuclear magnetic resonance spectroscopy. In the infrared method the AH stretching frequency is shifted to lower values and is accompanied by band broadening and increased intensity. Such changes are usually easily discernible, but in the case of very strong hydrogen bonds, such as (F
H
F)-, the shift and broadening is so drastic that it is difficult to assign the new frequencies correctly. In the case of nuclear magnetic resonance, hydrogen bonding usually shifts the proton resonance to lower fields. See also: Infrared spectroscopy; Nuclear magnetic resonance (NMR)
Neutron diffraction
While infrared and nuclear magnetic resonance techniques can yield considerable information about hydrogen bond formation, far greater information content is derived from diffraction studies of crystalline solids. The method of choice is neutron diffraction (versus x-ray diffraction), because in the former case hydrogen atoms scatter almost as well as any other atom while their scattering is often swamped out in the x-ray case. Neutron diffraction crystal structure analysis has become the best probe available for the study of the geometry of A H
Y bonds. Using this technique, it is generally observed that the A
H separation is ∼0.10 ± 0.01 nanometer and is much less than the H
Y separation; that is, hydrogen bonds are usually asymmetric. In the extreme case the hydrogen atom may be equally bonded to both atoms, as in certain O
H
O and (F
H
F)- containing systems where the atomic environment around A and Y is identical and symmetric. In such systems strong hydrogen bond formation is indicated when the A
Y separation is ∼0.02–0.03 nm less than the sum of the van der Waals radii. However, contrary to prior belief, even the shortest and strongest hydrogen bond known, (F
H
F)-, may be asymmetric. This was demonstrated in a neutron diffraction study of p-toluidinium hydrogen difluoride in which the two terminal F atoms exist in vastly different F
H
N hydrogen bonding environments (see illustration). The F
H distances are unequal, and the (F
H
F)- ion is asymmetric, because of the very different N
H
F hydrogen bonding environments around the F atoms. Thus it seems that the H atom of a strong (X
H
X)- bond is a probe of the X atom environment. Indeed, the hydrogen dichloride ion, (CHl2)-, with a bond energy of about 12 kcal/mole (49 kJ/mole) appears to be symmetric (centered H atom) in some salts and asymmetric in others. Other types of hydrogen atom interactions such as those of M
H
M and C
H
M, where M is a metal, are becoming increasingly important in catalysis. See also: Neutron diffraction
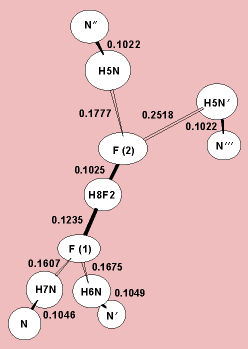


Theory
Developments in theory have made it possible to better define certain contributions to hydrogen bond energies. The relative importance of forces of different origin (dispersion, polarization, exchange, coulomb, and so on) have become possible to estimate by using both molecular orbital methods and perturbation theory. In general, it appears that quantum theory gives reliable descriptions of isolated imers and trimers, but fails when dealing with large clusters of the type found in condensed phases.