Key Concepts
The supporting tissues of an animal that serve to protect the body, or parts of it, and play an important role in physiology. The skeletal system, often termed skeleton, encompasses the anatomical structures composed of bone and cartilage, or a combination of both, which provide a framework for the body and serve as attachment for muscles. Skeletons can be divided into two main types based on the relative position of the skeletal tissues. When these tissues are located external to the soft parts, the animal is said to have an exoskeleton. If they occur deep within the body, they form an endoskeleton. All vertebrate animals possess an endoskeleton (Fig. 1), but most also have components that are exoskeletal in origin. Invertebrate skeletons, however, show far more variation in position, morphology, and materials used to construct them. See also: Bone; Cartilage; Muscle; Muscular system; Vertebrata
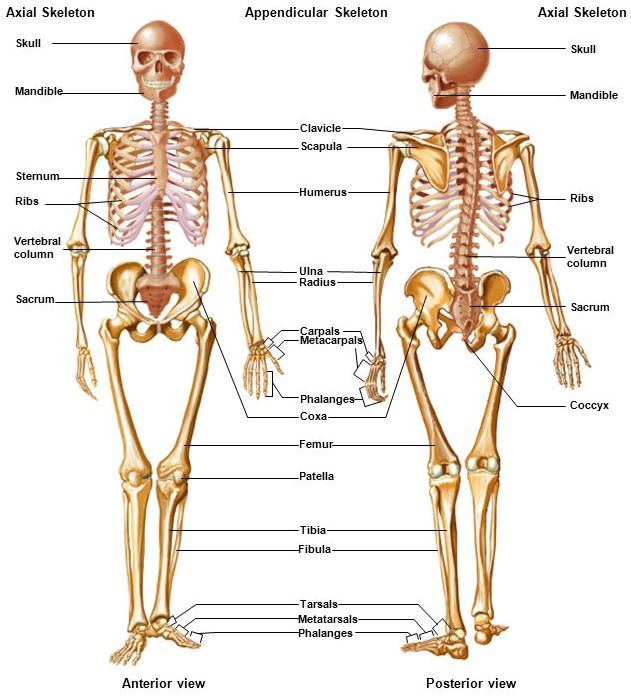
Exoskeletons
Many of the invertebrate phyla contain species that have a hard exoskeleton, including corals (Cnidaria); limpets, snails, and Nautilus (Mollusca); and scorpions, crabs, insects, and millipedes (Arthropoda). However, the exoskeletons of these invertebrates have different physical properties and morphologies. The form that each skeletal system takes presumably represents the optimal configuration for survival. See also: Arthropoda; Cnidaria; Mollusca
Calcium carbonate is the commonly found inorganic material in invertebrate hard exoskeletons. The stony corals have exoskeletons made entirely of calcium carbonate, which protect the polyps from the effects of the physical environment and the attention of most predators. Calcium carbonate also provides a substrate for attachment, allowing the coral colony to grow. However, it is unusual to find calcium carbonate as the sole component of the skeleton. It normally occurs in conjunction with organic material, in the form of tanned proteins, as in the hard shell material characteristic of many mollusks. Cuticular exoskeletons are widely distributed among the invertebrates. They are formed by proteins that have been stiffened by the chemical action of phenols (the process is called tanning). Mineral salts may be incorporated within the cuticle for additional strength and stiffness. See also: Calcite; Carbonate minerals
The major advantage of a hard exoskeleton is the high degree of protection afforded to the body organs against mechanical damage and desiccation. Some disadvantages are the relatively large mass of the skeletal structure in proportion to the size of the soft tissues, the inability of some animals to remodel the skeleton and repair damage, and problems for a growing animal bounded by rigid skeletal tissues. Arthropods have solved this last problem by periodically shedding the old skeleton (molting) and replacing it with a new, larger one.
Endoskeletons
Internal hard skeletons are less common among the invertebrates, but they are a feature of some mollusks and echinoderms. There are various degrees of skeletal reduction seen in these groups; for example, urchins have a complete rigid skeletal test formed of calcareous ossicles sutured together, and sea cucumbers possess a skeleton that is reduced to microscopic ossicles. The vertebrate endoskeleton (Fig. 2) is usually constructed of bone and cartilage; only certain fishes have skeletons that lack bone. In addition to an endoskeleton, many species possess distinct exoskeletal structures made of bone or horny materials. This dermal skeleton provides support and protection at the body surface. See also: Echinodermata
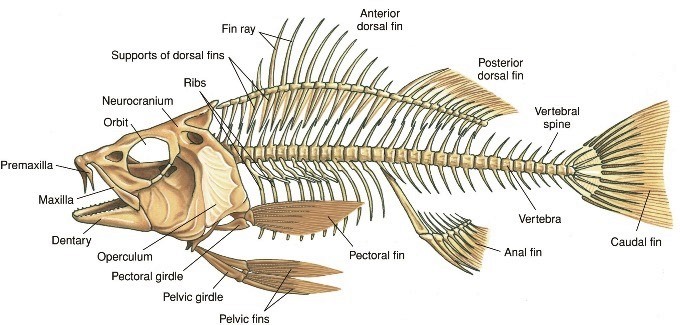
Human skeleton
Various structural components make up the human skeleton (Fig. 1), including collagen, three different types of cartilage (hyaline cartilage, fibrocartilage, and elastic cartilage), and a variety of bone types [woven, lamellar, trabecular (spongy), and plexiform (fibrolamellar)].
Collagen
Collagen is the most abundant protein in the body. It is of fundamental importance in all organ systems and is found in all parts of the musculoskeletal system. Tropocollagen (a structure composed of three polypeptide chains) forms the basic building unit of collagen. The rodlike tropocollagen molecules polymerize into large collagen fibrils. Cross-links between adjacent molecules and also between fibrils provide collagen with its tensile strength. In tendons and ligaments, these fibrils are bundled together to form larger fibers. Tendons are formed from parallel bundles of fibers; this arrangement allows tendons to support high uniaxial tensile loads to which they are subjected during activity. Although most ligament fibers are parallel, some have an oblique orientation. This reflects the more complex loading patterns applied to ligaments during movement, where small loads may be applied in a variety of directions. Collagen is stiff and strong only in tension. See also: Collagen; Ligament; Tendon
Cartilage
The formation of cartilage (chondrogenesis) from mesenchyme (the part of the mesodermal germ layer from which all connective tissues, blood vessels, blood, lymphatic system vessels, and the heart are derived) occurs in many areas of the embryo, such as the skull, limbs, and vertebral column. Most embryonic cartilage is replaced by true bone in endochondral bone formation; however, in many regions, the cartilage remains throughout life. The tissue consists of cartilage cells (chondrocytes) that manufacture, secrete, and maintain the extracellular organic matrix that surrounds them. This matrix contains a dense network of collagen fibrils within a concentrated solution of protein-polysaccharide molecules called proteoglycans. The three main types of cartilage are hyaline cartilage, fibrocartilage, and elastic cartilage. See also: Connective tissue
Hyaline cartilage, which is the most abundant form of cartilage in the body, ossifies during development to become bone. In the adult, it covers the articular surfaces of bones, supports the trachea and bronchi, forms the costal cartilages linking the first ten ribs to the sternum, and reinforces the nose. This type of cartilage contains extremely fine collagen fibrils. Hyaline articular cartilage is a special form found within synovial joints. Its material characteristics are perfectly adapted to the mechanically demanding environment of these joints.
Fibrocartilage is found predominantly in the pubic symphysis, the menisci of the knee, and the intervertebral discs. This type of cartilage is very durable and can withstand large tensile and compressive forces. It also represents a transitional material found at tendon and ligament insertions into bone and at the margins of some joint cavities. Histologically, it can be characterized by large collagen fibers running through the matrix.
Elastic cartilage is similar to hyaline cartilage, but contains abundant elastin fibers. These fibers give a yellowish appearance to the elastic cartilage and allow it to be very flexible, while still maintaining its strength. It is found in the outer ear, the auditory tube, and the larynx. See also: Elastin
Bone
Bone is a specialized rigid connective tissue. It comprises cells that produce an organic extracellular matrix, which consists of collagen fibers and ground substance, and inorganic materials in the form of mineral salts. In vertebrates, the mineral portion of bone consists primarily of calcium and phosphate in the form of hydroxyapatite crystals. The organic phase provides bone with its resilience and flexibility, whereas the inorganic phase allows the bone to be hard and rigid. The functions of bone are numerous, relating to the maintenance of mineral (mainly calcium) homeostasis, formation of blood cells, and mechanical requirements. In particular, bone has a protective function (Fig. 3), particularly with regard to the central nervous system. In addition, the skeleton is the supporting framework for the body and provides stiff levers on which muscles act to generate movement. See also: Calcium metabolism; Hematopoiesis; Homeostasis
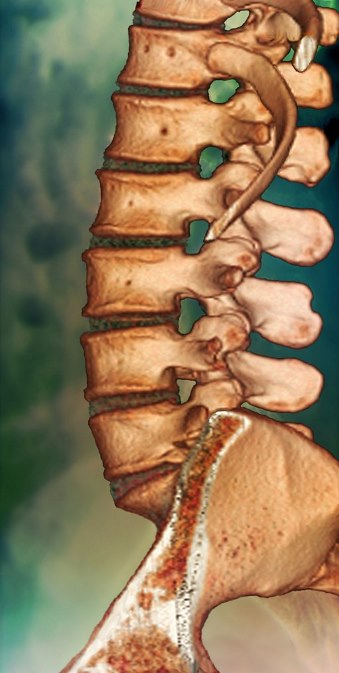
There are a number of bone types whose microstructure can be related to the mechanical functions required of the bone. The only type of bone that does not require a preexisting surface or structure for its formation is woven bone. Woven bone is formed during early stages of development; in adults, it is encountered only in pathological conditions, including bone fracture repair. It consists of randomly oriented, small-diameter, highly mineralized collagen fibers. It is not a strong form of bone because of its unordered structure and, particularly, because much of its volume is unmineralized. Its value lies in its ability to rapidly bridge the gap between broken ends of bone to act as temporary scaffolding during the process of repair. It is later removed by bone remodeling events.
Lamellar bone is the dense hard material that constitutes most of the skeleton. In a typical bone, such as the femur, lamellar bone has two distinct types of organization. In the shaft (diaphysis), the material is deposited in layers to form compact or cortical bone. A transverse section through the diaphysis would show a tree-ring-like arrangement of bone. Bone cells (osteocytes) occupy spaces between adjacent lamellae. They interconnect with vascular spaces and one another by fine cellular extensions running in narrow channels (canaliculi). These cells maintain the integrity and normal functioning of bone. Two other main cell types important in normal bone are the bone-forming cells (osteoblasts) and the bone-destroying (bone-resorbing) cells (osteoclasts).
Trabecular (spongy) bone is another form of lamellar bone found particularly in the expanded ends (epiphyses) of long bones and in the vertebrae. Although it is made of the same material as cortical bone (the dense outer surface of bone), the mechanical characteristics of trabecular bone differ as a result of the honeycomb arrangement of bone into interlacing struts (trabeculae). This spongy bone is less dense and stiff than cortical bone, and it has a large surface area that makes this tissue important for the exchange of calcium between the skeleton and the bloodstream.
Plexiform or fibrolamellar bone is a medium-density, relatively strong bone. It is found at sites of rapid growth where strength is also needed, such as in the limbs of large, fast-growing animals (for example, humans during the growth phase in puberty).
Development of the skeleton
All the components of the skeleton are derived from mesenchyme of either mesodermal or neural crest ectodermal origin. The majority of bones in the human body develop by the process of endochondral ossification; that is, the mesenchyme first forms a cartilaginous model that is subsequently replaced by true bone. The facial bones and certain bones of the cranium are formed by intramembranous ossification, in which mesenchyme is converted into skeletal elements by forming bone directly, without need of a cartilage stage. Sesamoid bones are specialized intramembranous bones that form within tendons.
Ossification
The development of bone from the embryo to the adult depends on the orderly processes of mitotic division, growth, and remodeling. These are determined largely by genetics, but are strongly influenced by hormonal action and nutrition. In endochondral ossification, the cartilaginous model is gradually calcified, resulting in cartilage cell (chondrocyte) death. Osteoblasts, together with a blood supply, invade the model and begin to secrete osteoid, which subsequently mineralizes and forms a primary ossification site. This process is repeated in the epiphyses of long bones to form secondary ossification sites. It is normal for the primary ossification site to form earlier, grow more rapidly, and cease ossification later than the secondary sites. The locations and ages at which ossification sites are active in the developing human skeleton are well documented.
Two parts of developing bones remain as cartilage: epiphyseal plates and articular cartilage. Epiphyseal plates are situated between the diaphysis and the epiphysis. Longitudinal bone growth occurs by chondrocyte proliferation on the diaphyseal sides. The plates are finally obliterated by an extension of diaphyseal ossification into these regions, thus preventing further growth in the length of the bone. Articular cartilage never ossifies, except in pathological situations such as osteoarthritis. See also: Arthritis
Changes in the radial dimensions of long bones occur by new material being deposited beneath the periosteum (a connective tissue membrane surrounding the bone). The endosteal membrane is the equivalent structure lining the internal surfaces of tubular bones. Bone tissue can be added or removed at either site by the action of osteoblasts or osteoclasts, respectively. During growth and aging, there is a tendency for net bone deposition periosteally, with net resorption endosteally.
Joints
In the human body, there are fibrous, cartilaginous, and synovial joints. Functionally, these joints can be considered as immovable (synarthrosis), partly movable (amphiarthrosis), and freely movable (diarthrosis) joints, respectively. See also: Joint (anatomy)
Immovable joints are represented by cranial sutures and epiphyseal plates prior to their ossification. Little or no movement is available at these joints. Instead, their primary function is to allow bone growth at their margin.
Partly movable joints include the pubic symphysis that joins the two halves of the pelvis anteriorly and the fibrocartilaginous intervertebral discs. Although the amount of movement between adjacent vertebrae is quite limited by disc stiffness, the vertebral column as a whole is quite flexible. Flexibility is achieved by combining each of the small movements permitted by individual discs.
Freely movable joints are the most complex and varied of the three types of joint, with their sizes and shapes matched to the functional requirements of the skeletal system at each location. At each joint, the surfaces of opposing bones are covered with a layer of articular cartilage that measures a few millimeters in thickness. The joint is enclosed within a flexible joint capsule, and the internal surface of this capsule is lined by the synovial membrane that secretes the lubricating synovial fluid into the joint space. The main functions of articular cartilage are to distribute compressive loads over a wide area in order to reduce contact stresses, and to allow relative movement of opposing joint surfaces with minimum wear and friction. The combination of articular cartilage and synovial fluid provides these joints with these remarkable properties.
Vertebrate skeletal anatomy
The vertebrate skeletal system consists of the axial skeleton (skull, vertebral column, and associated structures) and the appendicular skeleton (limbs or appendages). The basic plan for vertebrates is similar, although large variations occur in relation to functional demands placed on the skeleton.
Axial skeleton
The axial skeleton supports and protects the organs of the head, neck, and torso; in humans, it comprises the skull, ear ossicles, hyoid bone, vertebral column, and rib cage.
Skull
The adult human skull (Fig. 4) consists of 8 bones that form the cranium (braincase) and 13 facial bones that support the eyes, nose, and jaws. There are also 3 small, paired ear ossicles (the malleus, incus, and stapes) within a cavity in the temporal bone. The total of 27 bones represents a large reduction in skull elements during the course of vertebrate evolution. The main components of the skull are the neurocranium, dermatocranium, and visceral cranium. See also: Ear (vertebrate); Head
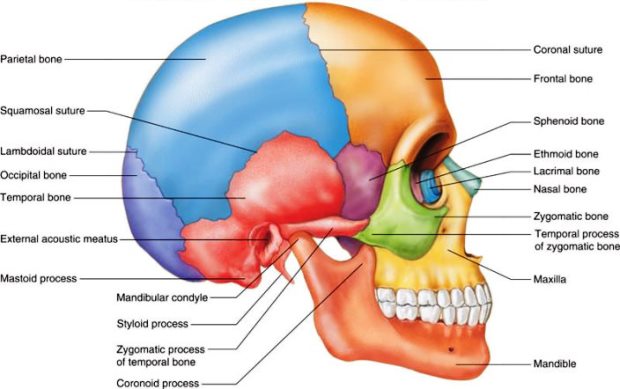
The brain and certain sense organs are protected by the neurocranium. All vertebrate neurocrania develop similarly, starting as ethmoid and basal cartilages beneath the brain, and as capsules partially enclosing the tissues that eventually form the olfactory, otic, and optic sense organs. Further development produces cartilaginous walls around the brain. Passages (foramina) through the cartilages are left open for cranial nerves and blood vessels. The largest opening is the foramen magnum, through which the spinal cord passes. Endochondral ossification from four major centers follows in all vertebrates, except the cartilaginous fishes. See also: Brain; Sense organ
Visceral skeleton
The visceral skeleton, that is, the skeleton of the pharyngeal arches, is demonstrated in a general form by the elasmobranch fishes, where all the elements are cartilaginous and support the jaws and the gills. The mandibular (first) arch consists of two elements on each side of the body: the palatoquadrates dorsally, which form the upper jaw, and Meckel's cartilages, which join ventrally to form the lower jaw. The hyoid (second) arch has paired dorsal hyomandibular cartilages and lateral, gill-bearing ceratohyals. This jaw mechanism attaches to the neurocranium for support. Variations in this articulation occur between species, effectively determining jaw movement and hence the feeding abilities of fishes.
Evolution of visceral cranium
In all jawed vertebrates, except mammals, an articulation between the posterior ends of the palatoquadrate and Meckel's cartilages (which may be ossified or ensheathed in bone) occurs between the upper and lower jaws. The bony fishes have elaborated on the primitive condition, where the upper jaw was fused to the skull and the lower jaw or mandible could move only in the manner of a simple hinge. Teleost fishes are able to protrude the upper and lower jaws. This motion, coupled with expansion of the buccal cavity, enables these fishes to generate the large suction forces used to draw food into the mouth. See also: Teleostei
In the course of mammalian evolution, the dentary of the lower jaw enlarged and a ramus expanded upward in the temporal fossa. This eventually formed an articulation with the squamosal of the skull. With the freeing of the articular bone and the quadrate from their function in jaw articulation, they became ear ossicles in conjunction with the columella, that is, a skeletal rod that formed the first ear ossicle. The remaining visceral skeleton has evolved from jaw and gill structures in the fishes to become an attachment site for tongue muscles and to support the vocal cords in tetrapods. See also: Mammalia; Tetrapoda
Vertebral column
The vertebral column (Fig. 3) is an endoskeletal segmented rod of mesodermal origin. It provides protection to the spinal cord, sites for muscle attachment, flexibility, and support, particularly in land-based tetrapods where it has to support the weight of the body. See also: Spinal cord
Hard, spool-shaped bony vertebrae alternate with tough (but pliable) intervertebral discs. Each typical vertebral body (centrum) has a bony neural arch extending dorsally. The spinal cord runs through these arches, and spinal nerves emerge through spaces. Bony processes and spines project from the vertebrae for the attachment of muscles and ligaments. Synovial articulations between adjacent vertebrae (zygapophyseal joints) effectively limit and define the range of vertebral motion. See also: Vertebra
Vertebral morphology differs along the length of the column. There are two recognized regions in fishes (trunk and caudal) and five in mammals (cervical, thoracic, lumbar, sacral, and caudal), reflecting regional specializations linked to function. Humans have 7 cervical, 12 thoracic, 5 lumbar, 5 (fused) sacral, and 4 coccygeal vertebrae. Most amphibians, reptiles, and mammals have 7 cervical vertebrae regardless of neck length (for example, giraffes have only 7), whereas the number is variable in birds. Specific modification to the first two cervical vertebrae in most reptiles, birds, and mammals provides the head with extra mobility. The presence of large ribs in the thoracic region often limits spinal flexibility. In typical tetrapods, the sacral region is usually modified for support of the pelvic girdle, whereas the number of caudal vertebrae varies greatly (from 0 to 50) between and within animal groups.
Ribs and sternum
Jawed fishes have ribs that help maintain the rigidity and support of the coelomic cavity. These ribs typically follow the connective tissue septa that divide successive muscle groups. In the caudal region, they are often small paired ventral ribs, fused on the midline to form the haemal arches. Ancestral tetrapods had ribs on all vertebrae, and their lengths varied between the vertebral regions. Modern amphibia (frogs and toads) have few thoracic ribs, and these are much reduced and never meet ventrally. Reptiles have varied rib arrangements, ranging from snakes with ribs on each vertebra (important for locomotor requirements) to turtles with only eight ribs, which are fused to the inside of the carapace. Flying birds (Fig. 5) and penguins have a greatly enlarged sternum that links the ribs ventrally. This provides a large surface area for the origin of the powerful pectoralis muscles that are used to power the downstroke of the wing. In humans, there are twelve pairs of ribs, which form a strong, but movable, cage encompassing the heart and lungs. See also: Amphibia; Aves; Reptilia
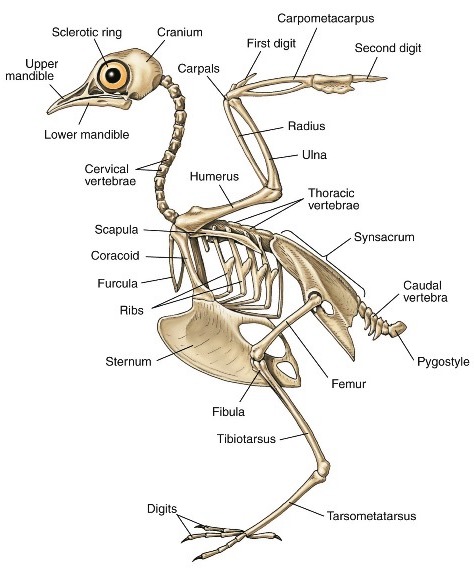
Appendicular skeleton
The appendicular skeleton comprises the pectoral and pelvic limb girdles and bones of the free appendages. The girdles provide a supporting base onto which the usually mobile limbs attach.
Pectoral girdle
The pectoral girdle has both endoskeletal and dermal components. The dermal components are derived from the postopercular dermal armor of primitive fishes and are represented by the clavicles and interclavicles in modern vertebrates, except where they are secondarily lost. Endochondral bone forms the scapula. In fishes, the main component of the girdle (the cleithrum) is anchored to the skull by other bony elements. Increased mobility of the girdle is seen in amphibia as it becomes independent of the skull. Further development and skeletal reduction have resulted in a wide range of morphologies, culminating in the paired clavicles and scapulae of mammals.
Birds have fused their paired clavicles and single interclavicle to form the wishbone or furcula. This provides an increased site for the origin of the pectoral muscles, both directly and from the fascial sheet spanning the two arms. In addition, clavicles have disappeared in certain groups of bounding mammals to allow greater movement of the scapula.
Pelvic girdle
The pelvic girdle forms by endochondral ossification, that is, the conversion of cartilage into bone. In fishes, it is a small structure embedded in the body wall musculature just anterior to the cloaca. Each half of the girdle provides an anchor and articulation point for the pelvic fins. In tetrapods, the girdle attaches to the vertebral column to increase its stability and assist in the support of body weight and locomotor forces. Humans, like all other tetrapods, have a bilaterally symmetrical pelvic girdle; each half is formed from three fused bones—the ischium, ilium, and pubis. A part of each of these elements forms the acetabulum (the socket-shaped component of the hip joint) that articulates with the femoral head. The human pelvis is bowl-shaped, helping to support the viscera in upright stance.
Paired fins and tetrapod limbs
Paired fins in fishes come in different forms, but all are involved in locomotion. In the simplest form, they are fairly rigid and extend from the body, functioning as stabilizers; however, they are also capable of acting like a wing to produce lift, as in sharks. In many fishes, the pectoral fins have narrow bases and are highly maneuverable as steering fins for low-speed locomotion. In addition, some fishes (such as the Australian lungfish) use their pectoral and pelvic fins to walk on the riverbed, whereas others have greatly enlarged pectoral fins that take over as the main propulsive structures (for example, rays and flatfish).
The basic mammalian pectoral limb consists of the humerus, radius, ulna, carpals, 5 metacarpals, and 14 phalanges; and the pelvic limb consists of the femur, tibia, fibula, tarsal, 5 metatarsals, and 14 phalanges. Variation in the shape and number of elements occurs primarily in the hand (manus) and foot (pes). A typical bird pelvic limb consists of a femur, tibiotarsus (formed by fusion of the tibia with the proximal row of tarsal bones), fibula, tarsometatarsus (formed by fusion of metatarsals II–IV), metatarsal I, and four digits (each consisting of 2–5 phalanges).
Locomotion and skeletal adaptations
Throughout evolution, the skeletal system has adapted to the needs of many different types of organisms. Such adaptations (Fig. 6) have been made for walking and running, speed, power, digging and burrowing, locomotion without limbs (as in snakes), and aerial and aquatic locomotion. For example, obvious modifications to the basic tetrapod skeleton have accompanied the acquisition of high-speed locomotion. The lengthening of limb segments allows for longer strides that, coupled with stride frequency, determine running speed. Limb elongation has been accompanied by a reduction in the number of skeletal elements in running specialists. Birds and bats are the only extant examples of vertebrates capable of continuous muscle-powered flight. See also: Flight
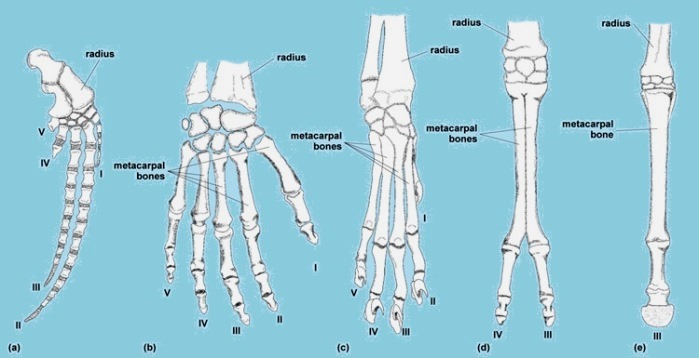
Birds have a highly modified forelimb complex (Fig. 5). The humerus, ulna, and radius have surface features linked to flight, but the real adaptation of these bones is their air-filled or pneumatic character. This reduces their mass and, therefore, the muscular work involved in moving the wings up and down. In addition, the major wing bones have relatively large diameters and are relatively thin-walled. These traits maximize torsional strength for any given mass of bone material, which is important for flying animals in which twisting forces on the wing skeleton are commonly encountered. Wing length is effectively determined by the lengths of the arm, forearm, and hand skeleton, but wing shape (breadth and sharpness of wing tips) is determined by feather morphology. Secondary adaptations to flight are seen in the pelvic girdle, with its extensive connection to the synsacrum presumed to be linked to absorbing the shock of landing.
Bats have adopted a different skeletal strategy. They use highly elongated bones (humerus, radius, metacarpals, and phalanges) to help support the whole wing (only the leading edge of the wing is supported in birds). These bones are not pneumatic, but contain marrow. Weight savings do occur in distal elements due to a reduction in mineralization. Large pectoral muscles attach to the ribs, and there is no massive, keeled sternum as in birds. Pelvic girdle reduction, thinner and straighter limb bones (which no longer have to resist large bending forces), partial loss of the fibula, and possession of large claws are all skeletal adaptations for a hanging lifestyle. See also: Chiroptera
Skeletal strength and size
Bones have to be stiff in order to act as levers for effective muscle action, and strong to resist failure. Although the form of the skeleton is genetically determined, the basic plan can be modified within limits by hormone action and the mechanical environment. See also: Biophysics; Hormone
The limb skeleton of terrestrial tetrapods is subjected to large dynamic forces during rigorous exercise. Even larger forces are applied to the skeleton by muscle action. Direct measurement of bone deformation under such conditions shows that the limb bones in most animals undergo similar levels of strain (that is, change in specimen length per original length) and are typically three to five times as strong as they need to be to resist failure. In addition, lamellar or cortical bone has the same mechanical properties whether taken from a mouse or an elephant. The strength of whole bones is thus a function of the amount of bone present and its geometric arrangement.
Furthermore, the bones of humans and elephants are proportionately more robust than those of mice. This occurs because a doubling in linear dimensions results in a fourfold increase in area and an eightfold increase in mass. If animals simply increased in size, it would result in the bones undergoing larger stresses in bigger animals. To keep bone stresses in large animals at the same level as in small animals, the bones must be made thicker.
Another obvious feature is that small animals adopt crouching (noncursorial) postures, whereas larger ones are typically cursorial, standing with straighter limbs. A more upright limb posture results in a better alignment of the forces generated during locomotion with the long axes of the bones. This reduces the forces that muscles need to generate to keep limbs extended, as well as the bending moments imposed on the bones. However, although cursorial postures lower stresses on the limb bones, they reduce agility.