Key Concepts
A multidisciplinary field concerned with the properties and uses of matter in terms of composition, structure, and properties. Materials science focuses on understanding how the properties (or characteristics) of materials are based on their atomic- and microscopic-scale structure. The term microstructure refers to this microscopic-scale structure. Materials science grew out of the fields of physical chemistry, polymer chemistry, and condensed-matter physics. See also: Physical chemistry; Solid-state physics
The engineering of materials involves the processing, testing, and selection of materials for various design applications (see illustration). Materials engineering grew out of the fields of metallurgy, plastics engineering, and ceramic engineering. See also: Metallurgy
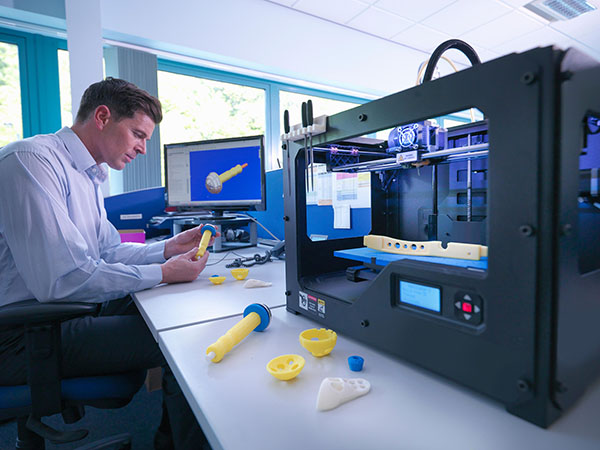
Types of materials
Most engineering designs incorporate a limited “menu” of materials, namely metals, polymers, and ceramics. As material properties are based on the structural arrangement of atoms, these categories follow directly from primary types of atomic bonding, namely metallic, covalent, and ionic.
Metals
Most of the elements in the periodic table exhibit atomic bonding that is metallic in nature. An alloy is a metal composed of more than one element. Copper and its alloys, such as brass (copper plus zinc), are common examples. The metallic bond involves a mobile “gas” of electrons that produces the electrical conductivity, thermal conductivity, and optical reflectivity characteristic of metals. The crystalline (regular and repeating) arrangement of atoms in metals generally provides attractive mechanical behavior in structural applications. Many alloys are reasonably strong and can be formed into practical shapes. See also: Alloy; Metal; Periodic table
The dominant examples are the iron-based or ferrous alloys, which include carbon and alloy steels and the cast irons. Although ferrous alloys represent the majority of metal applications in engineering designs, nonferrous alloys also are widely used. Major examples include alloys of aluminum, magnesium, titanium, and copper. Other examples include alloys based on zinc, lead (including solders alloyed with tin), refractory metals such as tungsten, and precious metals such as gold, platinum, and silver. See also: Ferroalloy; Iron alloys
Although most commercial metal alloys are crystalline in nature, rapid processing techniques can produce noncrystalline (amorphous) alloys. The resulting lack of periodic crystalline structure provides these amorphous alloys with some attractive properties, such as high strength combined with high deformability and excellent corrosion resistance.
Polymers
Polymers are composed of a few elements in the periodic table (usually carbon and hydrogen and possibly a few of the nonmetallic elements such as oxygen, nitrogen, fluorine, and silicon) that exhibit covalent atomic bonding. Polymers with carbon backbones (a carbon-carbon bonded chain) are the most common. A very common polymer is polyethylene (C2H4)n, where n is the degree of polymerization, a number around 1000 representing the number of ethylene monomers linked by covalent bonding. Other polymers can contain oxygen (for example, acrylics), nitrogen (for example, nylon), fluorine (for example, fluoropolymers), and silicon (silicones). See also: Polyacrylate resin; Polyamide resins; Polyfluoroolefin resins; Polymer; Polyolefin resins; Silicone resins
Polymers are generally good electrical insulators because directional covalent bonding between adjacent atoms usually does not provide “free” electrons for conduction. The use of polymeric insulation on electrical wires is a practical example. Polymers also can be optically transparent (for example, clear plastic wrap for food). The alternative name “plastic” comes from the extensive formability of many polymers during fabrication. See also: Dielectric materials
Polymers composed of high-molecular-weight molecules, such as (C2H4)n (for example, n > 100), often have a “spaghetti-like” structure, with weak secondary nonbonding interactions between molecules. The result is relatively low strength compared to metals. Nonetheless, many polymers have been engineered to have sufficient strength and stiffness which, when combined with their characteristically low density, allows them to be increasingly popular substitutes for traditional structural metals. Contemporary automotive design provides numerous examples of metal substitution by polymers.
Ceramics (and glasses)
Ceramics can be defined as nonmetallic and inorganic materials. Ceramics are chemical combinations of at least one metallic and at least one nonmetallic element (especially oxygen, with others possibly including carbon, nitrogen, phosphorous, and sulfur). Aluminum oxide (Al2O3) is a common example in which the metallic element aluminum is combined with the nonmetallic element oxygen. In fact, such chemical combinations are a fundamental tendency in nature, where metals chemically combine with nonmetallic elements from the environment. The rusting of iron is an example, both familiar and costly. Engineers also use similar environmental reactions to their advantage. Aluminum is a durable metal for structural applications because it can be anodized, a process in which a thin layer of aluminum oxide is produced on its surface. The aluminum oxide layer, in turn, usually protects the aluminum from further environmental degradation. See also: Ceramics
The ionic bond between metallic and nonmetallic atoms is a strong one. In aluminum oxide, the aluminum is a cation, Al3+, and oxygen is an anion, O2−. These oppositely charged ions are strongly attractive in an ionic bond. The thermal stability associated with the ionic bond makes ceramics resistant to high temperatures. The melting point of aluminum is 660°C (1220°F), while the melting point of aluminum oxide is 2020°C (3670°F). This heat resistance leads to the use of ceramics in refractory applications such as furnaces and boilers. See also: Refractory materials
Ceramics usually are crystalline in nature. Compared with metals and alloys, the greater structural complexity of ceramic structures leads to generally brittle mechanical behavior, limiting the applications of ceramics in many structural designs. See also: Brittleness
Although forming amorphous alloys is difficult, forming noncrystalline ceramics, called glasses, is relatively easy, as the crystallization of molten oxides can be a sluggish process. The most common examples are the silicate glasses, based on the mineral silicon dioxide (SiO2). After melting, many of the silicate materials can be cooled to a rigid state, while retaining the random atomic structure of the melt. Common window and container glasses are made in this way, with a typical composition (by weight) of roughly 75% SiO2, 15% Na2O, and 10% CaO. See also: Glass
A common theme in processing ceramics and glasses is the presence of porosity (in the form of tiny air bubbles). These small bubbles serve as light-scattering centers; for example, 3% porosity can turn a transparent material into a completely opaque one. The chalky appearance of many ceramics is the result. Porosity also weakens the material under structural loads.
Composites
Metals, polymers, and ceramics, which are fundamentally based on atomic bonding, cover the basic categories of structural materials. Engineers have developed many advanced materials based on the microscopic-scale combinations of them, resulting in a fourth category called composites. A common example is fiberglass, a composite of glass fibers (a few micrometers in diameter) embedded in a polymer matrix. In this case, the high strength of the small-diameter glass fibers is combined with the flexibility of the polymer matrix. Typical of structural composites, the fiberglass has properties superior to either component by itself. (Compared to fiberglass, the pure glass is more brittle and the pure epoxy is weaker.) More sophisticated composites include poly(p-phenylene terephthalamide) [Kevlar®] fibers and graphite fibers in various polymeric matrices, such as epoxy, polyester, polyetheretherketone (PEEKtm), and poly(phenylene sulfide) [PPS]. See also: Composite material; Metal matrix composite; Polymeric composite
Not all composites involve fiber reinforcement. Aggregate composites use particles embedded in a matrix. Concrete, with rock and sand in a calcium aluminosilicate (cement) matrix, is a common example. Tungsten carbide particles in a matrix of cobalt make excellent cutting tools. See also: Concrete
Semiconductors
Many structural materials can be classified adequately by the four categories described above. Materials also can be classified based on electrical conductivity rather than atomic bonding. Electrical classification provides a fifth category of materials—semiconductors. While metals are typically good electrical conductors and polymers and ceramics/glasses are typically electrical insulators, composites tend to have electrical properties that are the average of their individual components. Since the invention of the transistor at Bell Labs in 1948, covalently bonded semiconductors, with intermediate levels of electrical conductivity, have played an increasingly critical role in modern technology. The primary example is silicon, a central component of modern, solid-state electronics. Germanium is chemically similar to silicon and is a semiconductor widely used for electronic devices. Chemical compounds of elements close to silicon and germanium in the periodic table often display semiconduction. For example, gallium arsenide (GaAs) is a high-temperature rectifier and laser material. See also: Semiconductor
Advances in materials science and engineering
The field of materials science and engineering is a dynamic one, with new materials and processing technologies developed by materials scientists constantly being applied by materials engineers to new engineering designs. For example, 3D printing is now commonly used to print multiple materials (see illustration). Zero electrical resistance materials (superconductors) are used as thin-film filters for improved cellphone base stations. Micro-electro-mechanical systems (MEMS) include microscopic-scale gear systems fabricated by technologies developed for integrated circuits. Nanotechnology involves structures developed on the nanometer (10−9 m) scale, rather than the micrometer (10−6 m) scale. The resulting nanomaterials can display significantly improved mechanical, electrical, magnetic, thermal, and optical properties, compared to their counterparts on a coarser scale. See also: Micro-electro-mechanical systems (MEMS); Nanotechnology; Superconductivity; 3D printing
Microelectronics has steadily moved into the nanoscale, as critical components of integrated circuits are being produced at submicrometer dimensions (under 50 nanometers). Other developments include new forms of elemental carbon from fullerenes (such as C60 and carbon nanotubes) to graphene (one-atom-thick graphite sheets). These novel forms of carbon exhibit numerous, promising applications. See also: Carbon nanotubes; Fullerene; Graphene