Key Concepts
A region of spacetime exerting a gravitational field so strong that neither matter nor radiation can escape. Black holes are extreme cosmic objects predicted by German-born U.S. physicist Albert Einstein's theory of general relativity. Within a boundary known as the event horizon, the escape velocity needed to overcome the gravitational attraction of the black hole would exceed the speed of light, meaning that nothing that crosses over the event horizon can ever leave. Black holes are therefore by definition invisible, but because of their powerful gravitational fields, they can be indirectly observed through the highly conspicuous effects they have on their cosmic environment. These effects include the gravitational intake of matter through accretion disks, a process which generates tremendous heat and light and is well-observed at scales from binary star systems to the cores of galaxies. In the absence of ongoing accretion, black holes should also theoretically cause severe localized warping of spacetime, gravitationally lensing light from luminous sources and distorting their appearance (Fig. 1). The merging of two black holes each of about 30 times the Sun’s mass, detected in 2015 with the Laser Interferometer Gravitational-wave Detector (LIGO), opened a new and fruitful way of studying black holes, and revealed a mass range of stellar black holes greater than had been thought to be possible. See also: Astronomy; Escape velocity; Gravitation; Gravitational lens; Gravitational radiation; LIGO (Laser Interferometer Gravitational-wave Observatory); Relativity
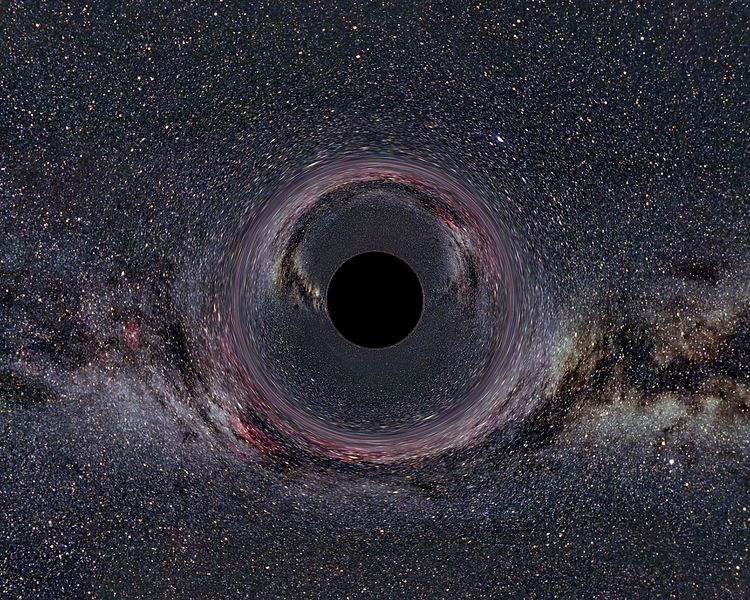
Black hole classes
In general, physicists recognize two broad classes of black holes, categorized by their masses. See also: Mass
Stellar black holes
Stellar black holes form from supergiant stars with an initial mass of approximately 10 times the mass of the Sun (solar masses) or more. When they reach the end of their lives, these colossal stars explode as supernovae, with a remaining core of approximately 3 solar masses collapsing into a black hole. Stars with a bit less initial mass collapse at the end of their lives into neutron stars that, although highly dense, do not pack as much matter into as small a volume as a black hole, and thus do not gravitationally create event horizons. Typical "dwarf" stars like the Sun evolve into remnants known as white dwarf stars. A stellar mass black hole can possess anywhere from a few to several dozens of solar masses. See also: Gravitational collapse; Neutron star; Star; Stellar evolution; Stellar population; Sun; Supergiant star; Supernova
Supermassive black holes
Supermassive black holes, found in the cores of nearly all galaxies, can contain anywhere from millions to billions of times the Sun's mass. The formation of supermassive black holes remains speculative. Hypotheses include the direct collapse of extremely massive gas clouds in the early universe into a likewise extremely massive "seed" black hole, which then voraciously consumed matter and merged with other black holes. This hypothesis explains how supermassive black holes could have formed, as they evidently have, within the relatively short time cosmic period of the first billion years following the big bang. Another hypothesis in this vein involves the formation of supermassive stars with tens of thousands of times the mass of the Sun, which then collapsed into supermassive black hole seeds. See also: Big bang theory; Galaxy; Galaxy formation and evolution; Searching for supermassive black hole signatures in galaxies; Supermassive star
Observation
Observations of black holes—which, by definition, are not directly detectable—rely on indirect methods using the gravitational interaction of the black hole with its surroundings. The first successful detection of a black hole in this manner involved the binary x-ray system Cygnus X-1 in 1971. These binary systems are composed of a massive, very dense object—a black hole or a neutron star—and a companion star. Because of strong gravitational forces, the companion star loses mass, which settles down into an accretion disk around the compact object (Fig. 2). Frictional forces cause the matter in the disk to spiral inward and also heat up the disk, causing it to emit thermal x-rays. See also: Binary star; Friction; X-ray astronomy; X-rays
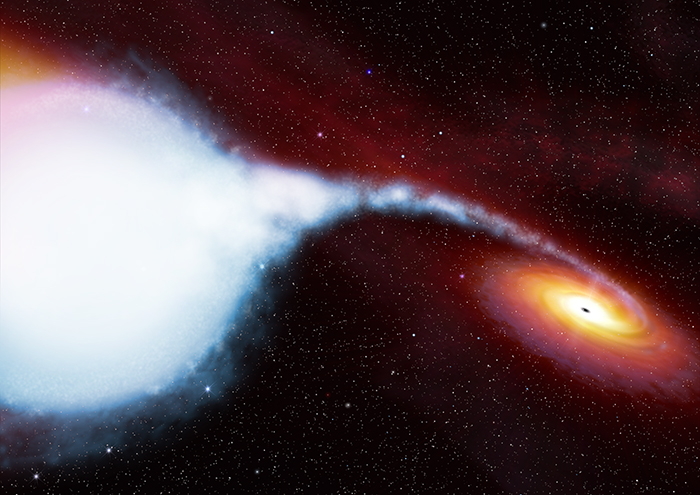
Whether the compact object is a neutron star or a black hole can be determined by analyzing the mutual motion of the components of the binary system and calculating the mass of the compact body. If the compact object (known to be compact because it is too dim to shine as brightly as a normal star) has more than about 5 solar masses, it must be a black hole. In the notable case of Cygnus X-1, the mass determination gives a value of about 10 solar masses, a value much larger than the maximum mass for a gravitationally stable neutron star and therefore strong evidence for the existence of a black hole in this system.
Another line of observational evidence for the existence of, in this case, supermassive black holes is drawn from active galactic nuclei (AGNs), whose luminosities are significantly higher than those of the largest nonactive galaxies. Variations in the luminosities of AGNs on time scales of tens of hours indicate a central engine whose diameter should be of the order of the product of the variation time scale and the speed of light. To generate observed AGN luminosities in a spherical volume defined by this diameter by nuclear-fusion processes like those taking place in stars, one would need such large quantities of matter that the gravitational potential energy would far exceed the nuclear energy. Therefore, the origin of the luminosity is attributed to the transformation of potential energy into radiation by the supermassive black hole as it devours matter from an accretion disk. Twin jets of material moving at near-light-speed blast outward from the black hole's poles as well; such astrophysical jets are commonly created by spinning objects, even much less massive ones such as protostars. The most luminous AGN are known as quasars; AGN whose jets are pointed directly at Earth are known as blazars. See also: High-energy astrophysics; Protostar; Quasar
Yet another way to indirectly detect supermassive black holes and infer their mass is by measuring the velocities of stars in their vicinity. This approach has informed calculations of the mass of the supermassive black hole at the center of our Milky Way Galaxy, associated with the radio source Sagittarius A* (pronounced "A star"). The measurements are made in the near infrared with adaptive optics on large telescopes. The orbits of the stars are Keplerian ellipses, which allow calculation of the mass of the central body with high precision. The calculations reveal a black hole mass of about 4 million solar masses. See also: Adaptive optics; Infrared astronomy; Milky Way Galaxy
Black holes and gravitational waves
A recently developed way of studying black holes and inferring their existence is through gravitational waves, also known as gravitational radiation. These are ripples in the spacetime fabric of the universe, initially predicted—as well as black holes themselves—by Einstein's theory of general relativity in 1915. Massive objects, such as black holes, should create gravitational waves when they accelerate. See also: Gravitational radiation; Relativity; Spacetime
The first instrument with the required sensitivity to detect the infinitesimal signatures of gravitational waves was LIGO. The instrument can detect displacements between mirrors, using interference properties generated by splitting laser beams into perpendicular directions, on the order of a ten-thousandth the diameter of a proton caused by gravitational waves passing through Earth. In 2015, LIGO detected the signature of two colliding black holes with 36 and 29 solar masses apiece—a mass range not previously observed for stellar black holes (Fig. 3). The merger did not generate electromagnetic radiation or the emission of any other particles, thus showing how gravitational wave astronomy will allow the study of previously inaccessible natural phenomena. Multiple gravitational wave detections since are advancing our understanding of black hole properties and the environments where they form. Though no specific environments are now known, and the current LIGO detections are of collisions billions of light-years away, eventually astronomers may find black holes in our galaxy in abundance in dense star clusters known as globular clusters. See also: Globular clusters; LIGO (Laser Interferometer Gravitational-wave Observatory); Photon; Star clusters
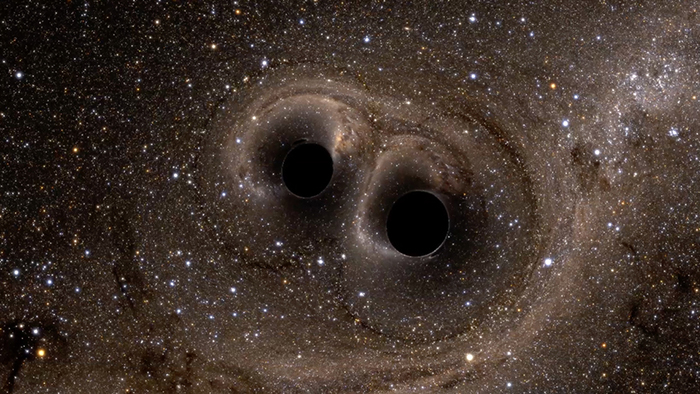
Since the 2015 detection of a black-hole merger, a neutron-star merger was detected not only in gravitational waves but also in light across the electromagnetic spectrum, ushering in a field known as multi-messenger astronomy. A highly anticipated gravitational wave-spawning event is the collision of a black hole with a neutron star, which should yield observable light and therefore offer further insights into compact objects, astrophysics, and fundamental physics. Future gravitational wave observatories are planned to seek the signals theoretically generated by sources including supermassive black hole mergers and from the earliest moments of the big bang. See also: Physics
Fate of black holes
According to general relativity, a finite mass can theoretically be compressed into a point of zero volume at the center of a black hole, creating an infinitely dense state of matter known as a singularity. The black-hole solutions of general relativity, ignoring quantum-mechanical effects as described later, are completely stable. Once massive black holes form, they should theoretically remain forever, and subsequent processes (for example, the accumulation of matter) only increase their size. Two black holes are able to coalesce and form a single, larger black hole, as LIGO has detected, but a single black hole could not split up into two smaller ones.
However, in 1974, English theoretical physicist and mathematician Stephen Hawking showed that when quantum effects are properly taken into account, a black hole should seem to emit thermal radiation. The radiation would result from the quantum-mechanical prediction that pairs of particles spontaneously form in the vacuum of space. These particles usually instantly annihilate each other, one being matter and the other being antimatter. Sometimes, though, a particle pair should form with one particle inside the black hole's event horizon and the other outside this boundary. The particle outside the event horizon would escape into space as so-called Hawking radiation, taking with it a tiny portion of the black hole's mass.
Over time, Hawking's hypothesis suggests that black holes can shrink and eventually disappear—so long as they are emitting Hawking radiation faster than they are accumulating matter or energy. Yet wherever in space a black hole might be located, it would receive significant, ambient radiation from other stars and the big bang itself through the cosmic microwave background. Because this ambient radiation is thought to be much larger than any emitted Hawking radiation, the black hole would not shrink. Even if the ambient radiation somehow did not reach the black hole, the time required for a typical black hole (formed from the collapse of a star) to completely decay is much longer than the age of the universe. The upshot: In the history of the universe, no stellar black holes have disappeared due to Hawking radiation. See also: Cosmic background radiation
The possibility remains that there are less massive black holes than those formed in stellar collapses. Theoretically, for instance, black holes of any mass could have been created at the beginning of the universe in the big bang. For smaller-mass black holes, the Hawking radiation process would be quite important. For example, a black hole created with mass of 1.72 × 1011 kg (3.8 × 1011 lb), about the mass of a large hill, would have radiated away all of its mass just recently (assuming that no mass had been accreted in the meantime). Black holes created with a mass smaller than this value would have disappeared earlier in the universe's history, and those with a larger mass would still exist.
The final stage of a black hole's evaporation would be quite violent and would take place quickly. As a black hole radiates, it loses mass, and its temperature rises. But a higher temperature means that the black hole radiates and loses mass at a faster rate, raising its temperature even further. The final burst, as the black hole gives up the remainder of its mass, would be a spectacular event. The final emission would contain all radiation and particles that could exist, even those not generated by existing accelerators.
At present, there is no evidence that points to the existence of black holes with small masses or having evaporated via a Hawking radiation process. Some physicists have suggested that the Large Hadron Collider—the most powerful particle accelerator built to date and that has operated since 2010—might produce miniature black holes that immediately evaporate due to Hawking radiation and thus be indirectly detectable. See also: Large Hadron Collider (LHC); Particle accelerator