Key Concepts
A sudden movement of the Earth caused by the abrupt release of accumulated strain along a fault in the interior. The released energy passes through the Earth as seismic waves (low-frequency sound waves), which cause the shaking. Seismic waves continue to travel through the Earth after the fault motion has stopped. Recordings of earthquakes, called seismograms, illustrate that such motion is recorded all over the Earth for hours, and even days, after an earthquake. Earthquakes are one of the most destructive natural phenomena and occur with very little warning (Fig. 1). As a result, earthquake early warning, or seismic alert, systems are being implemented in earthquake-prone cities and regions. See also: Earthquake early warning; Seismology
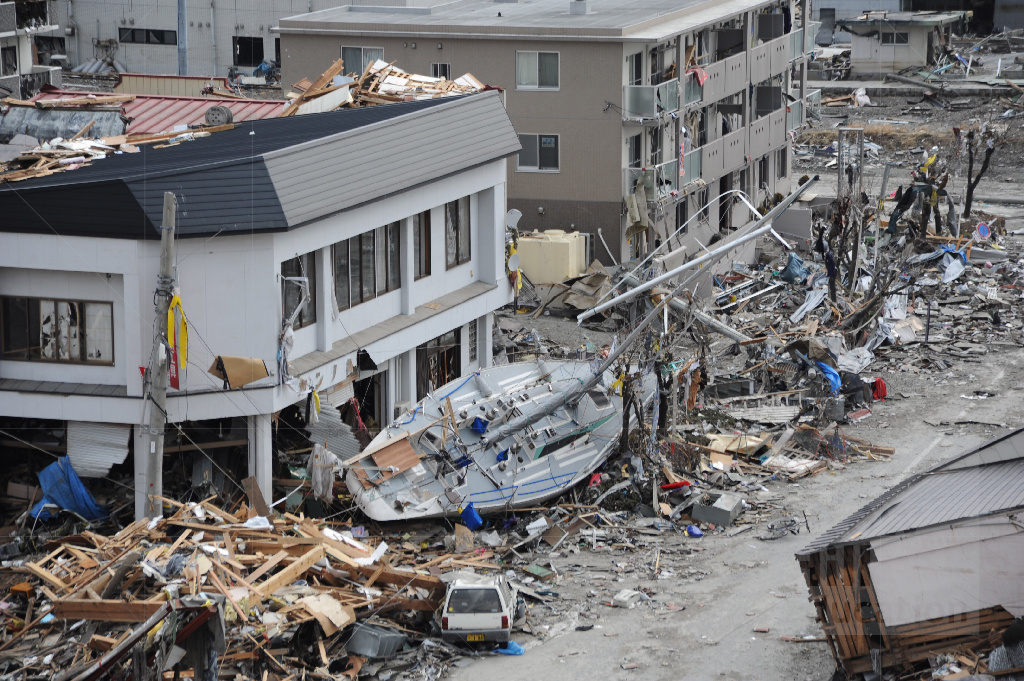
Characteristics
Earthquakes vary immensely in size, from tiny events that can be detected only with the most sensitive seismographs, to great earthquakes that can cause extensive damage over widespread areas. Although thousands of earthquakes occur every day, and have for billions of years, a truly great earthquake occurs somewhere in the world only once every year. When a great earthquake occurs near a highly populated region, tremendous destruction can occur within a few seconds. In 1976, 600,000 people were killed in Tangshan, China, by a single earthquake. The city of Lisbon, one of the principal capitals of that day, was utterly destroyed, with high loss of life, in 1755. Cities as Tokyo and San Francisco have been leveled by earthquakes. In these cases, much of the damage was not due to the shaking of the earthquake itself, but was caused by fires originating in the gas and electrical lines that interweave modern cities, and by damage to fire-fighting capability which rendered the cities helpless to fight the conflagrations.
Cause
The locations of earthquakes that occurred between 1900 and 2013 are shown on the map in Fig. 2. The map shows that earthquakes are not distributed randomly over the globe but tend to occur in narrow, continuous belts of activity. Approximately 90% of all earthquakes occur in these belts, which define the boundaries of the Earth's plates. The plates are in continuous motion with respect to one another at rates on the order of centimeters per year; this plate motion is responsible for most geological activity.
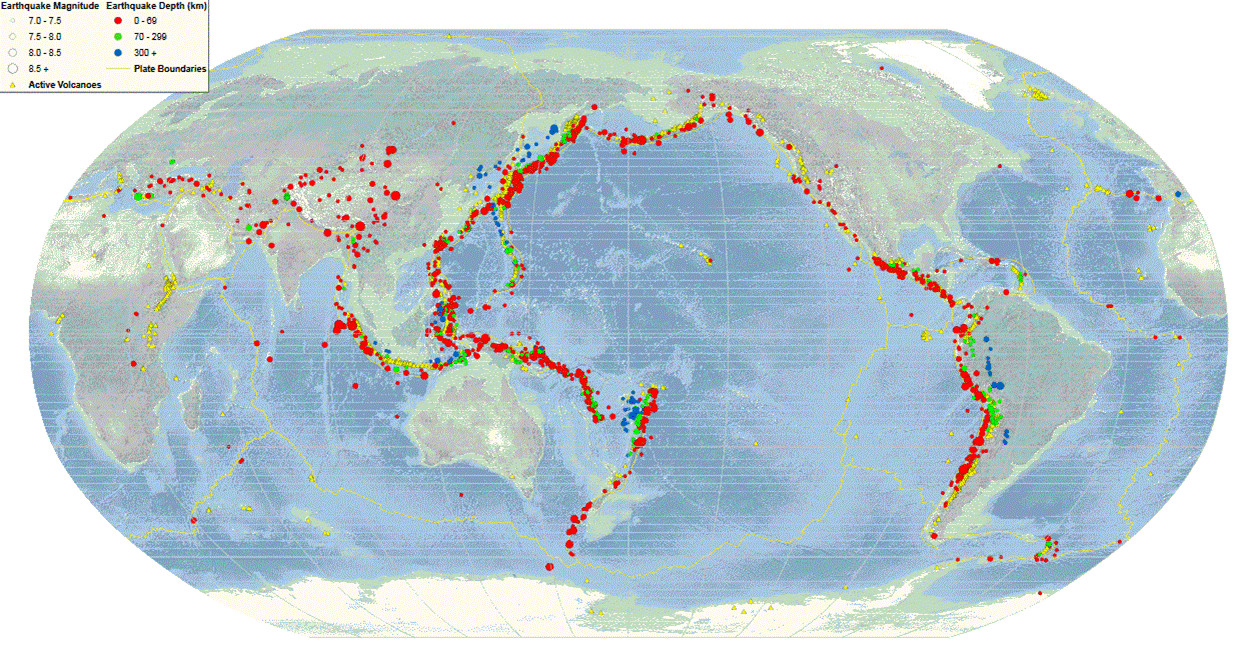
Plate motion occurs because the outer cold, hard skin of the Earth, the lithosphere, overlies a hotter, soft layer known as the asthenosphere. Heat from decay of radioactive minerals in the Earth's interior sets the asthenosphere into thermal convection. This convection has broken the lithosphere into plates which move about in response to the convective motion in a manner shown schematically in Fig. 3. The plates move apart at oceanic ridges. Magma wells up in the void created by this motion and solidifies to form new sea floor. This process, in which new sea floor is continually created at oceanic ridges, is called sea-floor spreading. Since new lithosphere is continually being created at the oceanic ridges by sea-floor spreading, a like amount of lithosphere must be destroyed somewhere. This occurs at the oceanic trenches, where plates converge and the oceanic lithosphere is thrust back down into the asthenosphere and remelted. The melting of the lithosphere in this way supplies the magma for the volcanic arcs which occur behind the trenches. Where two continents collide, however, the greater bouyancy of the less dense continental material prevents the lithosphere from being underthrust, and the lithosphere buckles under the force of the collision, forming great mountain ranges such as the Alps and Himalayas. Where the relative motion of the plates is parallel to their common boundary, slip occurs along great faults which form that boundary, such as the San Andreas fault in California. See also: Asthenosphere; Convection in the Earth; Earth's heat flow; Lithosphere; Magma; Volcanology
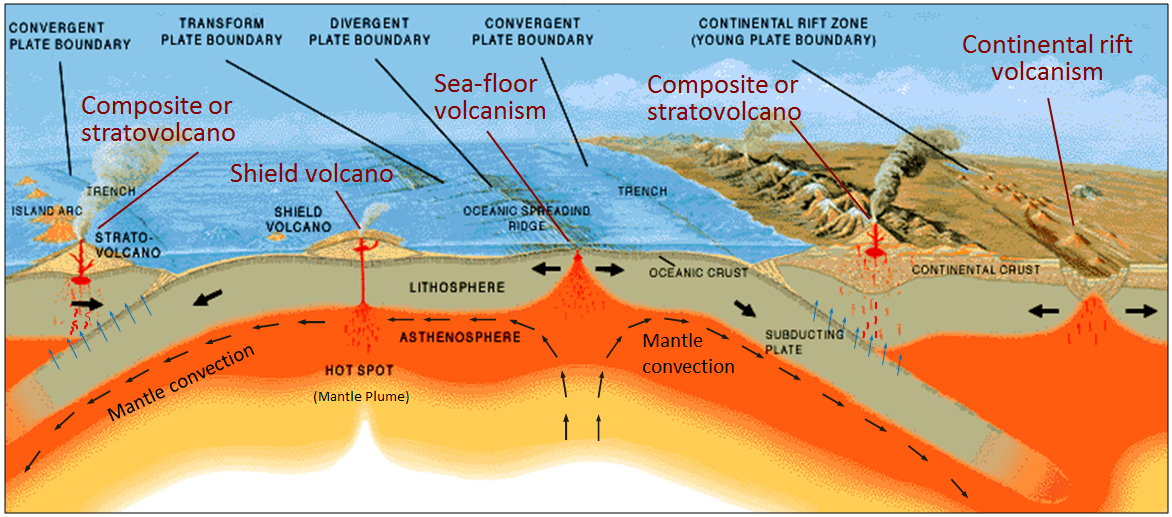
According to the theory of plate tectonics, the motion of the plates is very similar to the movement of ice floes in arctic waters. Where floes diverge, leads form and water wells up, freezing to the floes and producing new floe ice. The formation of pressure ridges where floes converge is analogous to the development of mountain ranges where plates converge. See also: Orogeny; Plate tectonics
Stick-slip friction and elastic rebound
As the plates move past each other, little of the motion at their boundaries occurs by continuous slippage; most of the motion occurs in a series of rapid jerks. Each jerk is an earthquake. It happens because, under the pressure and temperature conditions of the shallow part of the Earth's lithosphere, the frictional sliding of rock exhibits a property known as stick-slip, in which frictional sliding occurs in a series of jerky movements, interspersed with periods of no motion—or sticking. In the geologic time frame, then, the lithospheric plates chatter at their boundaries, and at any one place the time between chatters may be hundreds of years.
The periods between major earthquakes is thus one during which strain slowly builds up near the plate boundary in response to the continuous movement of the plates. The strain is ultimately released by an earthquake when the frictional strength of the plate boundary is exceeded. This pattern of strain buildup and release was discovered by H. F. Reid in his study of the 1906 San Francisco earthquake. During that earthquake, a 250-mi-long (400-km) portion of the San Andreas fault, from Cape Mendocino to the town of Gilroy, south of San Francisco, slipped an average of 12 ft (3.6 m). Subsequently, the triangulation network in the San Francisco Bay area was resurveyed; it was found that the west side of the fault had moved northward with respect to the east side, but that these motions died out at distances of 20 mi (32 km) or more from the fault. Reid had noticed, however, that measurements made about 40 years prior to the 1906 earthquake had shown that points far to the west of the fault were moving northward at a slow rate. From these clues, he deduced his theory of elastic rebound, illustrated schematically in Fig. 4. The figure is a map view, the vertical line representing the fault separating two moving plates. The unstrained rocks in Fig. 4a are distorted by the slow movement of the plates in Fig. 4b. Slippage in an earthquake, returning the rocks to an unstrained state, occurs as in Fig. 4c. See also: Fault and fault structures
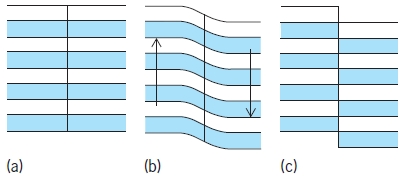
Classification
Most great earthquakes occur on the boundaries between lithospheric plates and arise directly from the motions between the plates. Although these may be called plate boundary earthquakes, there are many earthquakes, sometimes of substantial size, that cannot be related so simply to the movements of the plates.
Near many plate boundaries, earthquakes are not restricted to the plate boundary itself, but occur over a broad zone—often several hundred miles wide—adjacent to the plate boundary. These earthquakes, which may be called plate boundary–related earthquakes, do not reflect the plate motions directly, but are secondarily caused by the stresses set up at the plate boundary. In Japan, for example, the plate boundaries are in the deep ocean trenches offshore of the Japanese islands, and that is where the great plate boundary earthquakes occur. Many smaller events occur scattered throughout the Japanese islands, caused by the overall compression of the whole region. Although these small events are energetically minor when compared to the great offshore earthquakes, they are often more destructive, owing to their greater proximity to population centers.
Although most earthquakes occur on or near plate boundaries, some also occur, infrequently, within plates. These earthquakes, which are not related to plate boundaries, are called intraplate earthquakes, and can sometimes be quite destructive. The immediate cause of intraplate earthquakes is not understood. Some of them can be quite large. Three of the largest earthquakes known to have occurred in the United States were part of a sequence of intraplate earthquakes which took place in the Mississippi Valley, near New Madrid, Missouri, in 1811 and 1812. Another intraplate earthquake, in 1886, caused moderate damage to Charleston, South Carolina.
In addition to the tectonic types of earthquakes described above, some earthquakes are directly associated with volcanic activity. These volcanic earthquakes result from the motion of underground magma that leads to volcanic eruptions.
Sequences
Earthquakes often occur in well-defined sequences in time. Tectonic earthquakes are often preceded, by a few days to weeks, by several smaller shocks (foreshocks), and are nearly always followed by large numbers of aftershocks. Foreshocks and aftershocks are usually much smaller than the main shock. Volcanic earthquakes often occur in flurries of activity, with no discernible main shock. This type of sequence is called a swarm.
Size
Earthquakes range enormously in size, from tremors in which slippage of a few tenths of an inch occurs on a few feet of fault, to the greatest events, which may involve a rupture many hundreds of miles long, with tens of feet of slip. Accelerations exceeding 1 g (acceleration due to gravity) can occur during an earthquake. The velocity at which the two sides of the fault move during an earthquake is only 1–10 mi/h (10–100 cm/s), but the rupture front spreads along the fault at a velocity of nearly 5000 mi/h (3 km/s). The earthquake's primary damage is due to the generated seismic waves, or sound waves which travel through the Earth, excited by the rapid movement of the earthquake. The energy radiated as seismic waves during a large earthquake can be as great as 1012 cal (4.19 × 1012 joules), and the power emitted during the few hundred seconds of movement as great as a billion megawatts.
The size of an earthquake is given by its moment: average slip times the fault area that slipped times the elastic constant of the Earth. The units of seismic moment are dyne-centimeters. An older measure of earthquake size is magnitude, which is proportional to the logarithm of moment. Magnitude 2.0 is about the smallest tremor that can be felt. Most destructive earthquakes are greater than magnitude 6; the largest shock known was the 1960 Chile earthquake, with a moment of 1030 dyne-centimeters (1023 newton-meters) or magnitude 9.5. It involved a fault 600 mi (1000 km) long slipping 30 ft (10 m). The magnitude scale is logarithmic, so that a magnitude 7 shock is about 30 times more energetic than one of magnitude 6, and 30 × 30, or 900 times, more energetic than one of magnitude 5. Because of this great increase in size with magnitude, only the largest events (greater than magnitude 8) significantly contribute to plate movements. The smaller events occur much more often but are almost incidental to the process.
The intensity of an earthquake is a measure of the severity of shaking and its attendant damage at a point on the surface of the Earth. The same earthquake may therefore have different intensities at different places. The intensity usually decreases away from the epicenter (the point on the surface directly above the onset of the earthquake), but its value depends on many factors and generally increases with moment. Intensity is usually higher in areas with thick alluvial cover or landfill than in areas of shallow soil or bare rock. Poor building construction leads to high intensity ratings because the damage to structures is high. Intensity is therefore more a measure of the earthquake's effect on humans than an innate property of the earthquake.
Effects
Earthquake shaking produces many different effects. Although the fault motion that produced the earthquake is sometimes observed at the surface, often other earth movements, such as landslides, are triggered by earthquakes. On rare occasions the ground has been observed to undulate in a wavelike manner, and cracks and fissures often form in soil. The flow of springs and rivers may be altered, and the compression of aquifers sometimes causes water to spout from the ground in fountains. Undersea earthquakes often generate very long-wavelength water waves, which are sometimes called tidal waves but are more properly called seismic sea waves, or tsunami, which can be extremely damaging to coastal cities and ports. See also: Landslide; Tsunami
Prediction
Earthquake prediction research has been going on for nearly a century. A successful prediction, specifying the time, location, and magnitude of an earthquake, would save lives and billions of dollars in housing and infrastructure costs. Unfortunately, successful earthquake predictions are extremely rare. There are two basic categories of earthquake predictions: forecasts (months to years in advance) and short-term predictions (hours or days in advance). Forecasts are based a variety of research, including the history of earthquakes in a specific region, the identification of fault characteristics (including length, depth, and segmentation), and the identification of strain accumulation. Data from these studies are used to provide rough estimates of earthquake sizes and recurrence intervals.
An example of an earthquake forecast is the identification of seismic gaps, portions of the plate boundaries that have not ruptured in a major earthquake for a long time. These regions are most likely to experience great earthquakes in the future. Earthquake probability estimates are another example of forecasting. Geologic, geodetic, and seismic information are combined to estimate the frequencies of damaging earthquakes in a specific region. Regional earthquake probability estimate studies have resulted in forecasts of an 80–90% probability of a magnitude 7 or larger earthquake in the southern California region before 2024, and a 70% probability of a magnitude 6.7 or larger earthquake in the San Francisco Bay region before 2030.
Short-term earthquake prediction is still entirely in the realm of ongoing research, and no method is known to be reliable. Evidence is emerging that short-term prediction may be inherently impossible due to the complex and chaotic nature of the earthquake process.
Deep earthquakes
Most earthquakes occur at depths shallower than about 50 km (30 mi) and are usually found near plate boundaries. A few percent of all shocks occur at depths of 300–700 km (183–427 mi), depths that correspond to earth pressures of 100,000–250,00 atm (1–2.5 × 1010 Pa; Fig. 5). That the mantle can suddenly rupture rather than flow plastically at such conditions has elicited wonder since deep earthquakes were first discovered in the 1920s. Modern insight into these phenomena has come from scientific advances of plate tectonics, seismic tomography, and the mineral physics of the deep mantle based on very high pressure experiments on mantle minerals. See also: Earth's interior
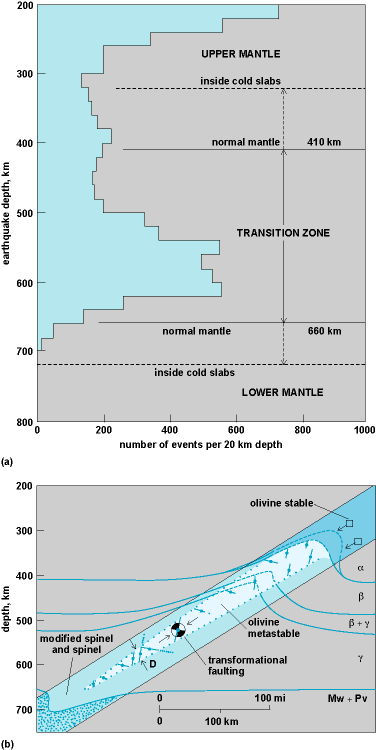
Most, if not all, deep earthquakes occur in inclined belts inside slabs, the cold, dense, and strong lithospheric plates that dive deeply into the Earth's mantle in places where plates are converging. Seismic waves have been used to image variations in the seismic wave speeds in the Earth. These anomalies in seismic tomographic images reflect differences in temperature, mineralogy, or composition. As expected, deep earthquakes occur in the high-wave-speed anomalies that mark cold slabs, anomalies that have been traced to depths as great as 2000 km (1220 mi) or more.
Curiously, earthquakes occur no deeper than 650–700 km (397–427 mi), far shallower than the maximum depths to which slabs descend. This abrupt shutoff and the gradual onset of the deep earthquake population at 300–350 km (183–214 mi) bracket approximately the transition zone of the mantle where seismic wave speeds abruptly increase (Fig. 5a). High-pressure experiments indicate that the mineralogy of the mantle changes at those depths and pressures from upper-mantle mineralogy (dominantly olivine and pyroxenes) to the minerals spinel, ilmenite, and majorite in the transition zone and, in turn, to the lower-mantle perovskite and oxide minerals. Slab mantle penetrating through the transition zone is expected to transform to these denser minerals. See also: Olivine; Oxide and hydroxide minerals; Perovskite; Pyroxene
Most deep earthquakes occur in the depth interval of the transition zone where upper-mantle slab minerals are reconstructed to their denser structural forms. Attention has therefore been drawn to the possibility that deep earthquakes are somehow caused by the mineralogical transformation of slabs as they descend into and through this region. Early speculation was that deep earthquakes represent rapid implosions that might occur when slab minerals transform suddenly to their denser, high-pressure forms. The patterns of seismic waves that radiate from deep earthquake sources indicate, however, that such disturbances represent slip on a fault, as do shallow earthquakes. If a connection exists between deep earthquakes and mantle phase changes, the underlying process must facilitate failure by faulting.
A clue to the nature of this possible connection comes from the observation that deep earthquakes do not occur in all slabs, only in those that are very cold because they are descending at very fast rates. Low slab temperatures are important because such conditions favor the metastable persistence of upper-mantle minerals in the coldest interiors of slabs as they descend into the transition zone (Fig. 5b). Laboratory studies show that some minerals deformed under metastable conditions will rupture by an unusual shear instability in which the mineral is transformed to denser minerals in the shear zone. This shear instability, called transformational faulting, is not inhibited by high pressures and hence is an attractive candidate for the faulting mechanism of deep earthquakes. According to this theory, deep earthquakes do not occur in the lower mantle because low-density upper-mantle slab rocks are too buoyant to sink into the lower mantle.