The surface manifestations of plumes, that is, columns of hot material, that rise from deep in the Earth's mantle. Hot spots are widely distributed around the Earth (Fig. 1). One of their characteristics is an abundance of volcanic activity which persists for long time periods (greater than 1 million years). When the lithosphere (the rigid outer layer of the Earth) moves over a plume, a chain of volcanoes is left behind that progressively increases in age along its length. Hot spots are believed to be fixed with respect to each other and the deep mantle so that the age and orientation of these chains provide information on the absolute motions of the tectonic plates. See also: Lithosphere; Plate tectonics
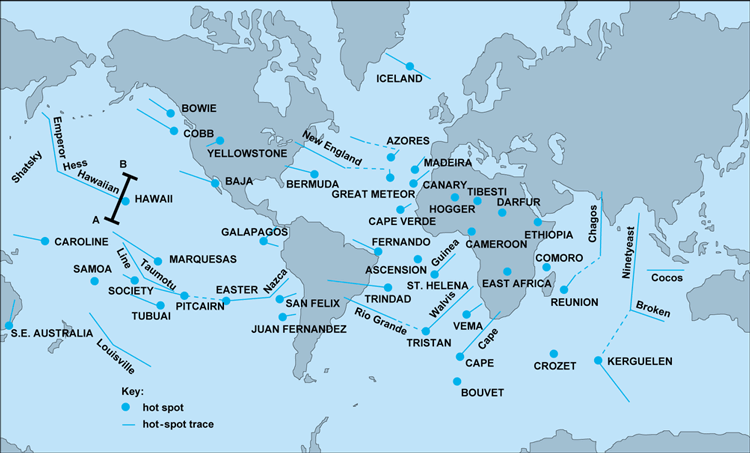
Volcanic expression
The Hawaiian-Emperor seamount chain in the central Pacific Ocean is a good example of a volcanic chain that was generated at a hot spot. The 3400-mi-long (5700-km) chain is made up mainly of tholeiitic lavas and ash tuff and pumice deposits. The lavas may have evolved from an initial submarine shield-building stage, through an explosive stage as they build up to sea level and finally to a subaerial posterosional stage. The largest volumes of lava formed during the shield-building stage, when extrusive processes built massive pillow and sheet lava flows, up to 3 mi (5 km) in height and 60 mi (100 km) in width, above the mean sea-floor depth. See also: Lava; Seamount and guyot
Not all hot-spot volcanism is expressed in terms of highly lineated, multistage, volcanic chains. The Réunion hot spot, for example generated the Deccan Traps, which extend laterally for thousands of kilometers over the Indian subcontinent. Large basalt provinces have also been mapped at sea: the seaward-dipping reflector sequences of the Norwegian and Greenland passive margins and the deep-water volcanic sill complexes of the eastern Atlantic and Pacific oceans are examples. Aseismic ridges that extend up to or close to the axes of mid-oceanic ridges are another example of hot-spot volcanism. When a hot spot (for example, Iceland) is centered on the axis, pairs of ridges such as the Iceland-Faeroes Rise and the Greenland Rise are formed. Sometimes the plate (for example, Africa) has migrated off the hot spot (such as Tristan da Cunha), leaving behind ridge systems that no longer extend to the ridge axis (such as Rio Grande Rise and Western Walvis). See also: Mid-Oceanic Ridge; Volcano
Topographic swells
Another characteristic of hot spots is their association with broad swells in the Earth's topography. At Hawaii, for example, the swell reaches widths of 900 mi (1500 km) and heights of up to 0.7 mi (1.2 km) above normal depths of the sea floor (Fig. 2). The Hawaiian hot-spot swell is believed to have been formed in response to either thermal or dynamic effects in an underlying mantle plume. The crustal and upper-mantle structure, which is constrained by seismic refraction data, shows that the oceanic crust is of uniform thickness beneath the swell. The long-wavelength correlation that is observed between the gravity anomaly and the topography (about 37 mGal mi-1 or 22 mGal km-1; Fig. 2) indicates that the mass excess of the swell is compensated by a low-density, high-temperature region below the crust. Swells of similar dimensions are found around Bermuda, Cape Verde, and Réunion. Some swells (for example, French Polynesia) are very large, reaching more than 1200 mi (2000 km) across, and may involve more than one hot spot (for example, Tubai, Society, Pitcairn, and possibly the Marquesas). The lack of a suitable reference complicates the identification of swells in continental regions. Swells similar in size to Hawaii have, however, been described around the volcanic centers of Dafur, Hoggar, and Tibesti in northern Africa. Others have been described from elsewhere in Africa and North America, where they may have influenced the development of sedimentary basins.
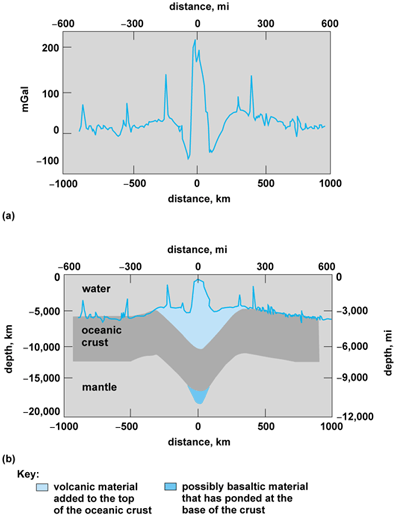
The uplift of hot-spot swells is believed to result from thermal perturbations in the underlying plume. The excess heights of swells suggest, on isostatic grounds, that temperature differences of about 450°F (250°C) occur between the plume and the surrounding mantle. Theoretical studies suggest that plumes are relatively narrow (about 180 mi or 300 km) when they begin their ascent through the mantle, but they widen to about 600–1200 mi (1000–2000 km) as they spread out beneath the lithosphere (Fig. 3). Hot ascending plumes may raise the temperature of the overlying lithosphere, thereby thinning it. The mechanism of thinning is not well understood, however, and it may involve either conductive heating of preexisting lithosphere or the removal of lithospheric material by some form of mantle flow.
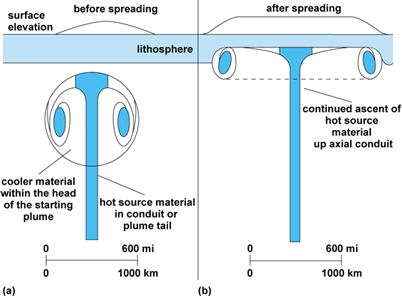
Models
Two classes of models have been proposed to explain hot-spot swells: the reheating and dynamic models. In the reheating model swell, uplift is produced by thermal expansion that is confined to the conducting portion of the lithosphere (the thermal boundary layer). In the dynamic model, however, there is a contribution to the uplift that is produced by vertical normal stresses exerted to the seismically defined base of the lithosphere (the mechanical boundary layer) by convection. Unfortunately, it is difficult to use surface observations to distinguish between these models. Geoid anomaly data suggest, for example, that the excess mass of swells is compensated by low-density, high-temperature material at depth. However, these data indicate only the depth of the greatest temperature differences. They are therefore indeterminate as to whether the temperature differences are confined to within the lithosphere as predicted by the reheating model, or whether they extend below as implied by the dynamic model.
The main distinguishing feature between the different uplift models is that the reheating model predicts a higher heat flow than the dynamic model. Discrimination between these models therefore depends on how the subsidence history, heat flow, and long-term strength (which is controlled mainly by the temperature) differ from those for unperturbed lithosphere of the same age. Many seamounts and oceanic islands show a subsidence history that follows closely what would be expected for the age of the lithosphere on which they were emplaced. Others, however, show a much greater subsidence. The subsidence history of Eniwetok and Bikini atolls in the Western Pacific, for example, is more typical of 25-million-year-old oceanic lithosphere than the 90-million-year-old lithosphere on which they were emplaced. This is supportive of a reheating model.
Heat-flow and elastic-thickness data, however, are not so easily explained by the reheating model. At Hawaii, for example, the measured heat flow is not significantly higher over the swell than the surrounding sea floor. Furthermore, the elastic thickness [a measure of the long-term (greater than 1-million years) strength of the lithosphere] is close to what would be expected on the basis of the tectonic-plate age. One explanation is that the perturbed heat has been stored in the lithosphere but has moved away with it as the Pacific plate (which is moving at rates greater than 100 mm yr-1) migrated over the Hawaiian plume. If this is so, the effects of reheating should be more apparent on slow-moving plates such as Africa, since there should been enough time for the plate to have been heated. The Cape Verdes, for example, have a relatively low elastic thickness (about 6 mi or 10 km less than expected), and high heat flow (about 20 mW m2 more than expected). These data can be explained if the 125-million-year-old oceanic lithosphere that underlies the Cape Verdes has been thermally reset by a hot spot to a thermal age of about 60–80 million years. The corresponding change in the depth of the sea floor, however, amounts only to about 1650–2700 ft (500–820 m), which is significantly less than the observed swell height of 6900 ft (2100 m).
The reheating model is therefore unable to explain the full height of the Cape Verdes swell, suggesting that other factors, such as dynamic effects, may be involved in its support. Finally, some hot-spot swells (for example, French Polynesia) show both normal and low elastic-thickness values. These observations are difficult to explain by either of the uplift models, suggesting instead a high degree of volcano individuality and the possibility of local rather than regional controls on some of the geophysical parameters that are used to characterize hot spots.
Chemical characteristics of volcanoes
In contrast to the chemically depleted basalts of the mid-oceanic ridges, hot-spot volcanoes have a higher proportion of those elements that tend to enter the liquid phase first when the solid mantle is heated, as compared to mid-oceanic ridge magmas that have experienced the same degree of crystal fractionation. This observation suggests that mid-oceanic basalts are derived from a mantle source that has previously undergone a melt extraction event or that the hot-spot magma source has been enriched in these so-called incompatible elements by some other mechanism. A higher concentration of radiogenic elements in hot-spot volcanoes also points to a magma source that is chemically distinct from that of mid-oceanic ridges. The chemical variation within hot-spot volcanoes, which reflects the heterogeneous nature of mantle sources and the interaction of chemically enriched melts with depleted mantle and crustal-derived melts, has been described in terms of a number of end-member types, which include depleted mantle, enriched mantle, and material with a component possessing a high ratio of uranium-238 to lead-204 (238U/204Pb) known as HIMU. One of the best known of the enriched mantle components, known as DUPAL, occurs in the French Polynesia superswell region, suggesting a link between them. HIMU components are also found within the superswell region. Possible sources for these components include subcontinental lithosphere (DUPAL) and subducted oceanic lithosphere (HIMU) now residing at the lower-upper mantle or core-mantle boundary that has become entrained in the convecting upper mantle before being involved in melting. See also: Earth interior
Material additions
Although the temperature differences in plumes are relatively small and imply small amounts of melting, the fact that mantle material is continually being fed through the melting region of the plume means that substantial volumes of magma can be generated by hot spots. Seismic refraction data, which indicate the amount of volcanic material that has been added to the top and bottom of the crust, indicate large variations in the production rates between hot-spot volcanoes. For example, rates that vary from 0.005 mi3 (0.02 km3) yr-1 for the Canary Islands to about 0.07–0.1 mi3 (0.3–0.5 km3) yr-1 for Réunion. The Canary Islands and Réunion were formed on old and young lithosphere, respectively, suggesting that the plume flux may depend on the thermal age of the overlying lithosphere: thick lithosphere inhibiting melt production, thin lithosphere encouraging it. One explanation of this observation is that plumes may be sites of decompression melting. If the lithosphere is thick, little melt is produced because insufficient decompression melting can occur. When the lithosphere is thin, a plume may develop beneath young oceanic lithosphere (that is, at a ridge crest), and then large quantities of melt can be produced. See also: Magma
There is a close association between magmatism and extension in the Earth's crust, such as continental margins that formed as a result of continental break-up. Some magmatism is to be expected at continental margins because of decompression melting. However, some margins (for example, Norway, Greenland, and Britain) are associated with such large thicknesses of volcanic material (up to 6 mi or 10 km) that they require high temperatures and high upwelling rates in order to explain them. Because of their association with Iceland, it has been proposed that the volcanic material at these so-called volcanic margins was generated at a hot spot. See also: Continental margin
An important problem is the relative timing of initial rifting and the volcanism. The earliest volcanism may occur as the plume is rising, and before rifting begins; but the volume of such volcanism is likely to be small. According to the decompression hypothesis, a bulk of the melting will follow initial rifting when the continental crust is thinned. See-floor spreading would therefore be expected to follow volcanism. Indeed, the separation of the Seychelles and Saya da Malha Banks from India followed the formation of the Deccan Traps at the Réunion hot spot 60–65 million years ago, and the opening of the North Atlantic followed the formation of the Brito-Arctic volcanic province at the Iceland hot spot 55–65 million years ago. The impingement of rising plumes on the lithosphere did not, however, lead to continental rifting in all cases (for example, Siberian Traps). Also, in some (for example, Karoo) there has been a long delay before rifting begins, suggesting that other controls may, in some cases, be more important.
Role of mantle plumes
Ever since W. J. Morgan introduced the concept of hot spots, there has been considerable discussion on the role of mantle plumes in the driving mechanism of plate tectonics. Early discussions were concerned with how ascending plumes interacted with convective motions in the mantle, especially the large-scale flow between mid-oceanic ridges and subduction zones. They now focus on whether hot spots play an active or passive role in the generation of plate motions. The uplift of plume-generated midplate swells may be sufficient, for example, to contribute to the forces that drive plate motions. However, plumes are passive, as is seen by their tendency to become entrained at mid-oceanic ridges (for example, Iceland) and large-offset fracture zones (for example, Louisville). See also: Subduction zones