Key Concepts
The synthesis of chemical compounds using light, especially the manufacture of organic compounds (primarily carbohydrates) from carbon dioxide and a hydrogen source (such as water), most often with simultaneous liberation of oxygen, by chlorophyll- or bacteriochlorophyll-containing cells. The term photosynthesis is used almost exclusively to designate one particularly important natural process: the use of light in the manufacture of organic compounds (primarily certain carbohydrates) from inorganic materials by chlorophyll- or bacteriochlorophyll-containing cells (Fig. 1). This process requires a supply of energy in the form of light because its products contain much more chemical energy than its raw materials. This is clearly shown by the liberation of energy in the reverse process, namely the combustion of organic material with oxygen, which takes place during respiration. See also: Carbohydrate; Chlorophyll; Energy metabolism; Plant respiration; Respiration
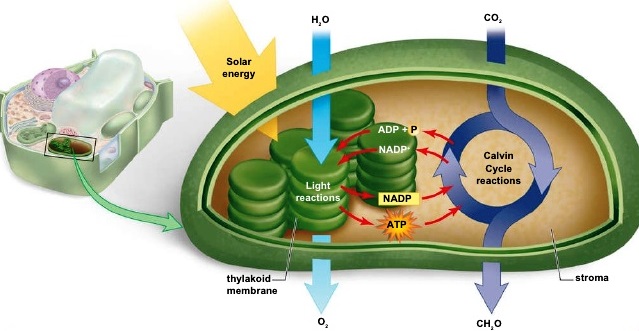
Among chlorophyll-containing plant and algal cells, as well as in cyanobacteria (formerly known as blue-green algae), photosynthesis involves the oxidation of water (H2O) to produce oxygen (O2) molecules, which are then released into the environment. This is called oxygenic photosynthesis (Fig. 2). In contrast, bacterial photosynthesis does not involve O2 evolution (production). In this case, other electron donors, such as hydrogen sulfide (H2S), are used instead of H2O. This process is called anoxygenic photosynthesis. See also: Algae; Cyanobacteria; Oxygen; Plant; Plant cell; Plant physiology; Water
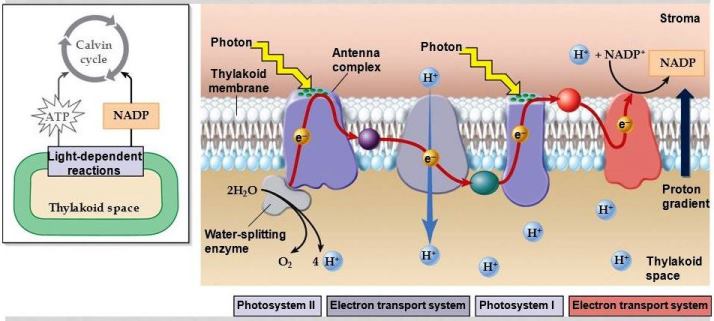
Because all types of photosynthesis require light, photosynthetic organisms are generally restricted to the narrow region of the Earth near the surface that receives sunlight. The only known exceptions are the anoxygenic photosynthetic bacteria that live near deep-sea hydrothermal vents and that utilize the very weak light given out by the hot vents. The light energy that is absorbed by the pigments of photosynthesizing cells, especially by chlorophyll or bacteriochlorophyll pigments, is efficiently converted into stored chemical energy. Together, the two aspects of photosynthesis—the conversion of inorganic matter into organic matter, and the conversion of light energy into chemical energy—combine to create the fundamental process of life on Earth: it is the ultimate source of all living matter and of almost all of the energy of life. See also: Hydrothermal vent
Oxygenic photosynthesis
The net overall chemical reaction of oxygenic photosynthesis (by plants, algae, and cyanobacteria) is shown in reaction (1):
(1)
where {CH2O} stands for a carbohydrate (sugar). The photochemical reaction in photosynthesis belongs to the type known as oxidation-reduction, with carbon dioxide (CO2) acting as the oxidant (electron acceptor) and water acting as the reductant (electron donor). The unique characteristic of this particular oxidation-reduction is that it is energetically unfavorable; that is, it converts chemically stable materials into chemically unstable products. Light energy is used to make this “uphill” reaction possible (Fig. 2). A considerable part of the light energy used for this process is stored as chemical energy. See also: Carbon dioxide; Oxidation-reduction
Temporal phases of photosynthesis
Photosynthesis is a complex multistage process that consists of nearly a hundred physical processes and chemical reactions. To make this complex process more understandable, it is useful to divide it into four temporal stages. Each phase is based roughly on the time scale in which it occurs. These phases are (1) photon absorption and energy transfer processes in antennas (or antenna chlorophylls, that is, molecules that collect light quanta); (2) primary electron transfer in photochemical reaction centers; (3) electron transport and adenosine triphosphate (ATP) formation; and (4) carbon fixation and export of stable products (Fig. 2). See also: Adenosine triphosphate (ATP)
Sites of photosynthesis
The photosynthetic process in plant cells and algae takes place within pigment-bearing subcellular organelles called chloroplasts (which are cell plastids) [Fig. 3]. In the leaves of the higher terrestrial plants, these organelles are usually flat ellipsoids measuring about 5 μm in diameter and 2.3 μm in thickness. Ten to 100 of them may be present in the average parenchyma cell of a leaf. Under the electron microscope, all chloroplasts show a layered structure with alternating lighter and darker layers of roughly 0.01 μm in thickness. These layers are membranes, called thylakoid membranes (thylakoid stands for a membrane sac), which contain proteins. These proteins bind all of the chlorophyll. The thylakoid membranes are the sites of the first three phases of photosynthesis. In algae, the number and shape of the chloroplasts are much more variable. See also: Cell plastids; Leaf; Parenchyma; Plant anatomy
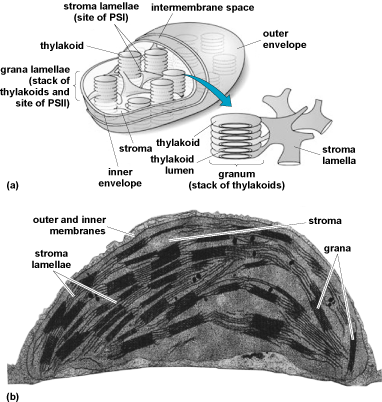
The photochemical apparatus is less complex in cyanobacteria. These cells are prokaryotes and therefore lack a nucleus and other organelles, including chloroplasts and mitochondria. The early phases of photosynthesis take place on thylakoid membranes, which extend throughout the interior of the cell. See also: Prokaryote
Two photosystems
Two photochemical events cooperate to carry out oxygenic photosynthesis (Fig. 2). Experiments suggest that plants contained two pigment systems. One (called photosystem I, or PSI; sensitizing reaction I) is primarily composed of chlorophyll a; the other (called photosystem II, or PSII; sensitizing reaction II) is also composed of chlorophyll a, but includes most of chlorophyll b or other auxiliary pigments (including the carotenoids and the phycobilins). Efficient photosynthesis requires the absorption of an equal number of quanta in PSI and in PSII. This ensures that the excitation energy within both systems is absorbed by the antenna system and partitioned to each photosystem, where the energy drives the chemical reactions. The PSII reaction is the one most closely associated with O2 evolution. The final result of this set of reactions is the oxidation of water to O2 and the reduction of a plastoquinone (an oxidation-reduction catalyst). Current evidence suggests that light absorbed by the major part of the accessory pigments is ultimately transferred to a special chlorophyll a molecule in the PSII reaction center, which is in a favorable position to act as an energy trap.
The two photochemical events (PSI and PSII) take place on four large protein complexes embedded in the thylakoid membrane (Fig. 4). Note that the membrane has an inherent asymmetry in that the protein complexes are oriented in a particular way in the membrane. This orientation is essential to the proper functioning of photosynthesis.
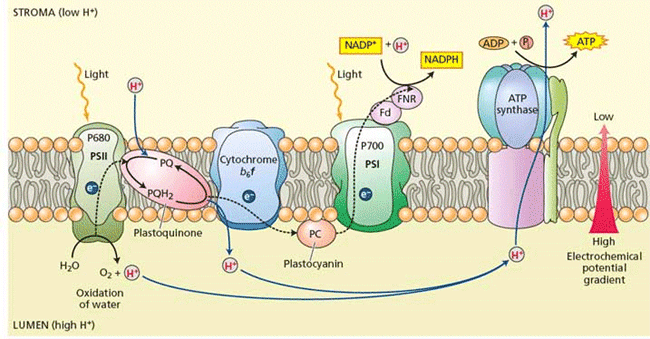
Figure 5 shows the Z scheme, which delineates the way in which the two photosystems cooperate to carry out the electron transfer reactions involved in photosynthesis. It is an energetic diagram, in that the energy of the component, which is measured as the midpoint redox potential Em , is shown on the y axis and the progress of the reaction is shown on the x axis. The two vertical arrows in the diagram represent energy input to the system due to photon absorption. See also: Photon
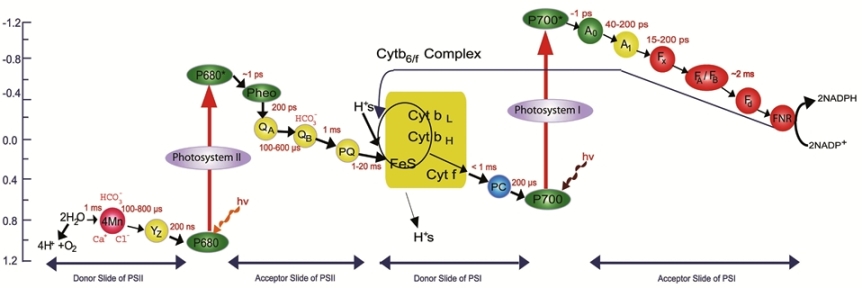
Photophosphorylation
When illuminated in the presence of adenosine diphosphate (ADP) and inorganic phosphate (Pi), the inner cytoplasmic membranes from photosynthetic bacteria, cyanobacterial cells, and chloroplasts from green plants and algae use light energy to synthesize adenosine triphosphate (ATP). About 42 kilojoules (kJ) of converted light energy in this reaction is stored in each mole of the high-energy phosphate, ATP. This photophosphorylation is coupled with energy-releasing steps in photosynthesis, such as the electron flow from PSII to PSI. When phosphorylation is associated with noncyclic electron flow from H2O to NADP+, it is called noncyclic photophosphorylation. In addition, under certain conditions, electrons in PSI, instead of going to NADP+, may return to an intermediate (such as a cytochrome, plastoquinone, or plastocyanin) and thus close the cycle. This type of cyclic electron flow, mediated by added cofactors as well as ADP and inorganic phosphate, also leads to the production of ATP and has been termed cyclic phosphorylation; it has been shown to exist under certain experimental conditions in vivo. See also: Nicotinamide adenine dinucleotide (NAD)
Accessory antenna pigments
Besides chlorophyll a (which is present in nearly all oxygenic photosynthetic organisms), there are other chlorophylls, including chlorophyll b in the green algae and higher plants. In addition, chlorophyll c replaces chlorophyll b in brown algae, whereas most of the chlorophyll a in the marine cyanobacterium Acaryochloris marina is replaced by chlorophyll d. There are also nonchlorophyllous pigments belonging to two groups: the carotenoids and the phycobilins. The carotenoids (named because of their similarity to the orange pigment of carrots) are a variable assortment of pigments found in all photosynthetic higher plants and algae. The phycobilins, or vegetable bile pigments, are chemically related to animal bile pigments. They are either red (phycoerythrin) or blue (phycocyanin). All of these pigments are associated with specific proteins to form so-called antenna pigment proteins. The structures of many of these antenna complexes are known, and their absorption spectra have been analyzed (Fig. 6). See also: Carotenoids; Phycobilin
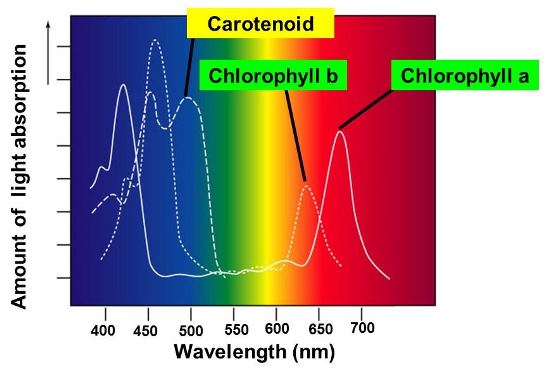
Carbon dioxide fixation
The light-dependent conversion of radiant energy into chemical energy as adenosine triphosphate (ATP) and reduced nicotinamide adenine dinucleotide phosphate (NADPH) serves as a prelude to the utilization of these compounds for the reductive fixation of CO2 into organic molecules. Such molecules, broadly designated as photosynthates, are usually (but not invariably) in the form of carbohydrates (for example, glucose polymers or sucrose) and form the base for the nutrition of all living things, as well as serving as the starting material for fuel, fiber, animal feed, oil, and other compounds used by humans. Collectively, the biochemical processes by which CO2 is assimilated into organic molecules are known as the photosynthetic dark reactions, which are so named because light is not required (in contrast to the photosynthetic light reactions). Notably, CO2 fixation by photosynthetic organisms is an important mechanism by which this “greenhouse” gaseous molecule is removed from the atmosphere during carbon cycling on Earth. Approximately 100 pentagrams of carbon (1 pentagram equals 109 metric tons) as CO2 is assimilated annually into organic molecules by photosynthesis (about half of this amount is assimilated by photosynthetic marine algae).
C3 photosynthesis
The essential details of C3 photosynthesis can be seen in Figure 7. The entire cycle can be separated into three phases—carboxylation, reduction, and regeneration. For the purposes of understanding, it is easiest to start with three molecules of CO2 because the smallest intermediate in the cycle consists of three carbons. During the initial carboxylation phase, the three molecules of CO2 are combined with three molecules of the five-carbon compound ribulose 1,5-bisphosphate (RuBP) in a reaction catalyzed by the enzyme RuBP carboxylase/oxygenase (Rubisco) to form three molecules of an intermediate, unstable enzyme-bound six-carbon compound. These unstable molecules are hydrolyzed further into six molecules of the three-carbon compound phosphoglyceric acid (PGA). These products of the carboxylation phase, that is, the six (three-carbon) PGA molecules, are phosphorylated by six molecules of ATP (releasing ADP to be used for photophosphorylation via the light reactions) to form six 1,3-bisphosphoglycerate (1,3-BP) molecules. The resulting compounds are reduced (that is, in the reduction phase of the C3 cycle) by the NADPH formed in the photosynthetic light reactions to form six molecules of the three-carbon compound phosphoglyceraldehyde (PGAL). PGAL is isomerized to form another three-carbon compound, dihydroxyacetone phosphate (DHAP). PGAL (the aldehyde) and DHAP (the ketone) are energetically equivalent, reduced compounds and can be considered to be the products of the reductive phase of the C3 photosynthetic cycle. PGAL and DHAP together form the triose phosphate (TP) pool of the chloroplast. The chloroplast TP pool is primarily composed of PGAL (the isomerase responsible for PGAL:DHAP interconversion favors PGAL formation).
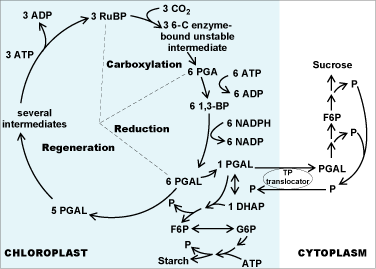
The rest of the C3 photosynthetic cycle (the regeneration phase) involves enzymatic steps that allow regeneration of RuBP, the initial carboxylation substrate. One molecule of PGAL is made available for combination with DHAP isomerized from a second PGAL (requiring a second “turn” of the Calvin-Benson-Bassham cycle wheel) to form a six-carbon sugar. The other five PGAL molecules, through a complex series of enzymatic reactions, are rearranged into three molecules of RuBP, which can again be carboxylated with CO2 to continue the cycle.
It should be noted that the enzyme RuBP carboxylase/oxygenase (Rubisco) [Fig. 8] that incorporates CO2 into an organic compound also allows O2 to react with RuBP—hence the "oxygenase" in the name. This reaction initiates the process called photorespiration, which results in the release of one previously incorporated molecule of CO2 for every two molecules of O2 that are allowed to react. Due to its low catalytic efficiency, Rubisco can be up to half of the soluble protein in C3 chloroplasts, and it is likely the most abundant protein found in nature. Structurally, Rubisco is a large and complex enzyme, comprising eight large polypeptide subunits and eight small subunits. See also: Enzyme; Photorespiration
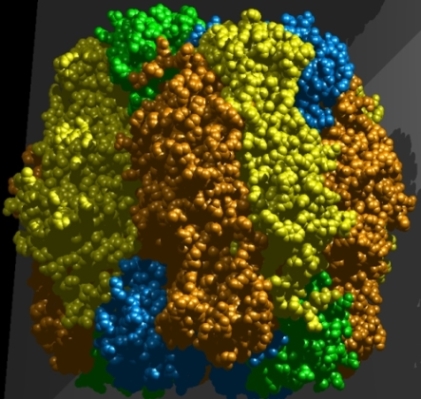
The net product of two “turns” of the cycle, that is, a six-carbon sugar (G6P or F6P), is formed either within the chloroplast in a pathway leading to starch (a polymer of many glucose molecules) or externally in the cytoplasm in a pathway leading to sucrose (condensed from two six-carbon sugars, glucose and fructose). This partitioning of newly formed photosynthate leads to two distinct pools; starch is stored in the photosynthesizing “source” leaf cells, and sucrose is available either for immediate metabolic requirements within the cell or for export to “sinks”, including developing reproductive structures, roots, or other leaves. Factors within the photosynthesizing cell, such as energy requirements in different compartments (mitochondria, cytoplasm, and chloroplasts), along with energy needs of the plant (for example, increased sink requirements during different developmental stages), and external environmental factors (for example, light intensity and duration) ultimately regulate the partitioning of the newly formed photosynthetic product (PGAL) into starch or sucrose. See also: Plant metabolism
C4 photosynthesis
Initially, the C3 cycle was thought to be the only route for CO2 assimilation, although it was recognized by plant anatomists that some rapidly growing plants (such as maize, sugarcane, and sorghum) possessed an unusual organization of the photosynthetic tissues in their leaves (Kranz morphology). Further work demonstrated that plants having the Kranz anatomy utilized an additional CO2 assimilation route, which is now known as the C4-dicarboxylic acid pathway (Fig. 9). Carbon dioxide enters a mesophyll cell, where it is combined (in the form of bicarbonate) with the three-carbon compound phosphoenolpyruvate (PEP) via the enzyme PEP carboxylase to form a four-carbon acid, oxaloacetate, which is reduced to malic acid or transaminated to aspartic acid. The four-carbon acid moves into bundle sheath cells, where the acid is decarboxylated and the CO2 reassimilated via the C3 cycle. To complete the cycle, the resulting three-carbon compound, pyruvic acid, moves back into the mesophyll cell and is transformed into PEP (at the cost of 2 ATP molecules) via the enzyme pyruvate phosphate dikinase located in the mesophyll chloroplasts. The net effect of this cycle is to increase the CO2 concentration around Rubisco, thereby reducing photorespiration via the competing oxygenase activity of this enzyme.
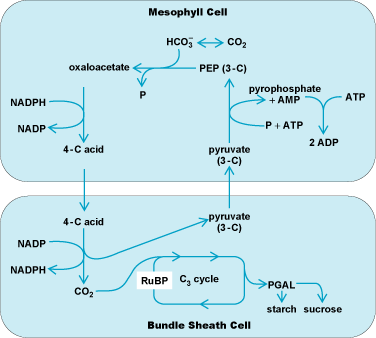
C4 metabolism is classified into three types, depending on the primary decarboxylation reaction used with the four-carbon acid in the bundle sheath cells. The majority of C4 species (exemplified by sugarcane, maize, crabgrass, and sorghum) belong to type 1 and employ NADP-malic enzyme (NADP-ME) for decarboxylation. NAD-malic enzyme (NAD-ME) C4 plants belong to type 2 and include Amaranthus, Atriplex, millet, pigweed, and purslane; in contrast, C4 plants categorized as type 3 use phosphoenolpyruvate carboxykinase (PCK) for decarboxylation and include Panicum grasses.
Crassulacean acid metabolism (CAM) photosynthesis
Under arid and desert conditions, where soil water is in short supply, transpiration during the day when temperatures are high and humidity is low may rapidly deplete the plant of water, leading to desiccation and death. By keeping stomata closed during the day, water can be conserved; however, the uptake of CO2, which occurs entirely through the stomata, is prevented. Therefore, many desert plants (including those in the Crassulaceae, Cactaceae, and Euphorbiaceae families) have evolved, apparently independently of C4 plants, a similar strategy of concentrating and assimilating CO2 by which the CO2 is taken in at night when the stomata open; in general, water loss is low because of the reduced temperatures and correspondingly higher humidities. The biochemical understanding of the mechanisms involved in this process was first studied in plants of the Crassulaceae family; thus, the process has been called crassulacean acid metabolism (CAM). See also: Plant-water relations
In contrast to C4 photosynthesis, where two cell types usually cooperate, the entire CAM process occurs within an individual cell; the separation of C4 and C3 is thus temporal rather than spatial. At night, CO2 combines with PEP through the action of PEP carboxylase, resulting in the formation of oxaloacetic acid and its conversion into malic acid. The PEP is formed from starch or sugar via the glycolytic route of respiration. Thus, there is a daily reciprocal relationship between starch (a storage product of C3 photosynthesis) and the accumulation of malic acid (the terminal product of nighttime CO2 assimilation) [Fig. 10].
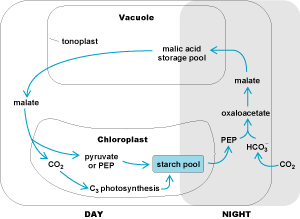
Bacterial photosynthesis
Certain bacteria have the ability to perform photosynthesis. A general equation for bacterial photosynthesis is shown in reaction (2):
(2)
where A represents any of a number of reductants, most commonly S (sulfur). Because photosynthetic bacteria cannot use water as the hydrogen donor, they are incapable of evolving oxygen. They are therefore called anoxygenic photosynthetic bacteria. (Note that the prokaryotic cyanobacteria are excluded because their photosynthetic system closely resembles that found in eukaryotic algae and higher plants.) Anoxygenic photosynthetic bacteria can be classified in four major groups: (1) Proteobacteria, including nonsulfur purple bacteria (Rhodospirillaceae) and sulfur purple bacteria (Chromatiaceae); (2) green sulfur bacteria (Chlorobiaceae); (3) green gliding bacteria (Chloroflexi); and (4) Heliobacteria (Heliobacteriaceae). Like plants, algae, and cyanobacteria, anoxygenic photosynthetic bacteria are capable of photophosphorylation, which is the production of adenosine triphosphate (ATP) from adenosine diphosphate (ADP) and inorganic phosphate (Pi) using light as the primary energy source.
Photosynthetic bacteria do not have specialized organelles such as the chloroplasts of green plants. Electron micrographs of certain photosynthetic bacteria show tiny spherical sacs, with double-layered walls, as a result of invaginations that form stacks of membranes. Other photosynthetic bacteria have invaginations that form thylakoids. These intracytoplasmic membranes, often called chromatophores, contain the photosynthetic apparatus and can be isolated easily by mechanical disruption of bacteria followed by differential centrifugation. Isolated chromatophores are often used for biochemical and biophysical studies of bacterial photosynthesis. See also: Chromatophore
The pigment bacteriochlorophyll (BChl) is a necessary component for bacterial photosynthesis. There are specialized BChl molecules in bacteria that engage in the primary chemical reactions of photosynthesis. In addition to these specialized molecules, there are 40–50 BChl molecules referred to as antenna pigments, whose sole function is to harvest light energy and transfer it to reaction center molecules. This is similar to the photosynthetic unit of plants, algae, and cyanobacteria. Each reaction center contains a special pair (dimer) of BChl molecules that engage in chemical reactions after they trap the absorbed light energy. They are also called the energy traps of bacterial photosynthesis. See also: Bacterial physiology and metabolism