Key Concepts
The succession of events that culminates in the asexual reproduction of a eukaryotic cell; also known as the cell division cycle. In a typical cell cycle (Fig. 1), the eukaryotic parent cell doubles its volume, mass, and complement of chromosomes, then sorts its doubled contents to opposite sides of the cell, and finally divides in half to yield two genetically identical offspring. Implicit in the term cycle is the idea that division brings the double-sized parent cell back to its original size and chromosome number, and ready to begin another cell cycle. This idea fits well with the behavior of many unicellular organisms; however, for multicellular organisms, the daughter cells may differ from their parent cell and from each other in terms of size, shape, and differentiation state. See also: Cell (biology); Cell biology; Cell differentiation; Cell division; Chromosome; Eukaryota; Genetics
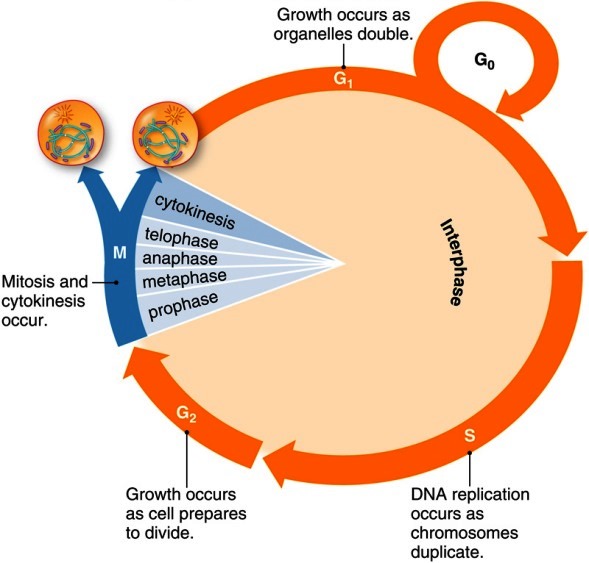
The time required for completion of a eukaryotic cell cycle varies enormously from cell to cell. Embryonic cells that do not need to grow between divisions can complete a cell cycle in as little as 8 min, whereas cycling times of 10–24 h are typical of the most rapidly dividing somatic cells (that is, the cells of the body of an organism except the germ cells). Many somatic cells divide much less frequently: liver cells divide about once a year, and mature neurons never divide. Such cells may be thought of as temporarily or permanently withdrawing from the cell cycle.
Eukaryotic phases
The cell cycle is divided into two main parts: interphase and M phase (Fig. 1). During interphase, the cell grows and replicates its chromosomes. Interphase accounts for all but an hour or two of a 24-h cell cycle, and is subdivided into three phases: gap phase 1 (G1), synthesis (S), and gap phase 2 (G2). Interphase is followed by M phase, which consists of mitosis (nuclear division) and cytokinesis (cell division). This relatively brief part of the cell cycle includes some of the most dramatic events in cell biology. See also: Mitosis
G1 phase
Gap phase 1 (G1) begins at the completion of mitosis and cytokinesis and lasts until the beginning of S phase. This phase is generally the longest of the four cell cycle phases and is quite variable in length. During this phase, the cell chooses either to replicate its deoxyribonucleic acid (DNA) or to exit the cell cycle and enter a quiescent state (the G0 phase). Late in G1 phase, the cell becomes committed to replicating its DNA. In mammalian cells, the time at which this commitment occurs is called the restriction point. See also: Deoxyribonucleic acid (DNA)
S phase
Replication of the chromosomes is restricted to one specific portion of interphase, called S phase (DNA synthesis phase), which typically lasts about 6 h. In mammalian cells, the start of S phase (the actual initiation of DNA synthesis) takes place several hours after the cell has committed to carrying out DNA synthesis. During S phase, each chromosome replicates exactly once to form a pair of physically linked sister chromatids. In animal cells, a pair of centrioles is also duplicated during S phase. See also: Centriole; Sister chromatid cohesion
G2 phase
The portion of interphase that follows S phase is called gap phase 2 (G2). Some cells can exit the cell cycle from G2 phase, just as they can from G1 phase.
M phase
M phase includes the overlapping processes of mitosis and cytokinesis. Mitosis is divided into five stages: prophase, prometaphase, metaphase, anaphase, and telophase. During prophase, the chromosomes condense and the football-shaped mitotic spindle begins to form. Prometaphase begins when the nuclear envelope abruptly disappears and the chromosomes begin to migrate toward the spindle's midline. When the chromosomes reach the midline, the cell is said to be in metaphase. Metaphase ends and anaphase begins when the sister chromatids abruptly separate from each other and move toward the spindle poles. During telophase, the nuclear envelope reforms around each set of chromosomes, the chromosomes decondense, and mitosis is completed. Cytokinesis (Fig. 2) usually begins during anaphase and ends at a point after the completion of mitosis. At the end of cytokinesis, the parent cell has formed its two G1 phase progeny, and the cell is ready to repeat the cycle. See also: Mitosis and the spindle assembly checkpoint
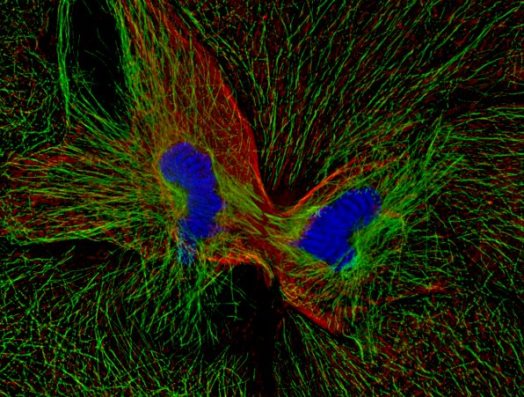
Control of cell cycle
The network of proteins that regulate DNA synthesis (G1/S), mitotic entry (G1/M), and mitotic exit (the transition from mitotic metaphase to anaphase and then out of mitosis) appears to be well conserved throughout eukaryotic evolution. At the heart of these cell cycle transitions is the periodic activation and inactivation of cyclin-dependent protein kinases. In addition, in multicellular eukaryotes, pathways regulating entry into and exit from the cell cycle entrain these central cyclin-dependent kinases to extrinsic signals.
Cell cycle entry
In multicellular eukaryotes, most cells spend most of their time in the quiescent G0 state. In response to peptide growth factors, cells initiate a signal transduction cascade that culminates in entry into G1 phase. Components of these mitogenic signaling pathways include receptor tyrosine kinases [such as the epidermal growth factor (EGF) receptor], small G-proteins (such as Ras), and signal-relaying protein kinases (such as MAP kinase).
Many components of these mitogenic signaling pathways are capable of causing malignant transformation when overexpressed or inappropriately activated. The cancer-causing forms of these proteins are termed oncoproteins, and their genes are termed oncogenes. Conversely, several tumor suppressor genes (which can promote malignant transformation when inactivated) have been shown to encode proteins that oppose mitogenic signaling proteins. These discoveries underscore the idea that cancer may result when the biochemical machinery that controls normal cell growth goes awry. See also: Cancer; Oncogene; Tumor suppressor gene
Mitotic control
When interphase cells are artificially fused with cells in mitosis, the nuclei of the interphase cells rapidly enter mitosis. This discovery indicates that some dominant mitosis-inducing factor is present in M-phase cells. Studies on Xenopus frog oocytes and eggs, unusually large cells that are naturally arrested in G2 phase (oocytes) or meiotic M phase (eggs), underscore this point: when oocytes are microinjected with cytoplasm from eggs, the oocytes enter M phase, a process termed oocyte maturation. The factor responsible, maturation promoting factor (MPF), is present and active during both meiotic M phase and mitotic M phase, and can be found in M-phase cells from evolutionarily distant organisms. This suggests that MPF is the universal M-phase trigger. See also: Meiosis
Other crucial insights into M-phase control came from studies of growth regulation in the yeast Schizosaccharomyces pombe. Mutations in genes that regulate M-phase onset of S. pombe give rise to organisms that are too long or too short. Once such a mutant strain is isolated, the gene responsible for its altered size can be identified by molecular genetic methods. Two genes identified through this approach were cdc2+ and cdc13+ . Genes related to cdc2+ and cdc13+ were found in a variety of other eukaryotes, suggesting that they encoded universal regulators of M-phase onset. See also: Molecular biology; Mutation
Ultimately, it was realized that the biochemical studies of M-phase regulation in frog oocytes and the genetic studies of M-phase regulation in S. pombe had succeeded in identifying the same M-phase regulators. MPF proved to be a complex of the Xenopus homologs of S. pombe cdc2+ and cdc13+ (the Cdc13 protein is usually called a B-type cyclin because it rises and falls in abundance during the cell cycle). The fact that two very different approaches and two evolutionarily distant organisms had converged upon the same M-phase regulators underscored the importance and universality of the regulators.
The Cdc2/cyclin B complex is essential for initiation of all M phases in all organisms. The complex functions as a protein kinase (an enzyme that adds a phosphate group to specific amino acid residues in target proteins). The catalytic subunit of the complex is Cdc2; cyclin B is necessary for the activation of Cdc2 and is responsible for localizing the complex to the nucleus at the onset of M phase.
At the onset of anaphase, a protein complex termed the anaphase-promoting complex (APC) becomes activated, bringing about the proteolytic destruction of several key mitotic regulators. One critical APC target is cyclin B; its destruction is required for mitotic exit. APC also directly or indirectly causes the destruction of other proteins (for example, securins and cohesins) that keep the pairs of sister chromatids attached to each other and keep the cell in metaphase. As was the case with MPF, the rapid progress in the understanding of APC function and the appreciation of its universality were made possible through a combination of genetic studies (in the budding yeast Saccharomyces cerevisiae) and biochemical studies (mostly in frog egg extracts).
G1- and S-phase control
The other cell cycle transitions, for example, the commitment to DNA synthesis that occurs in G1 phase, and the initiation of DNA synthesis at the start of S phase, are also triggered by activation of heterodimeric protein kinases consisting of Cdc2-like catalytic subunits (termed Cdks, for cyclin-dependent kinases) and cyclin-like regulatory subunits. Thus, the entire cell cycle may be driven by the sequential activation and inactivation of various Cdk/cyclin complexes (Fig. 3).
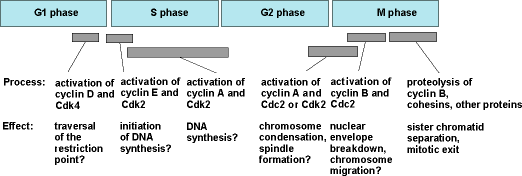