Key Concepts
A linear polymer made up of a specific sequence of deoxyribonucleotide repeating units linked by 3′,5′-phosphodiester bonds and acting as the carrier of genetic information. Deoxyribonucleic acid (DNA) [Fig. 1] is the common structure that forms the basic building blocks of life. The set of DNA molecules that contains all genetic information for an organism is called its genome. DNA is found primarily in the nuclei of eukaryotic cells and in the nucleoid of bacteria. Small amounts of DNA are also found in organelles [including mitochondria and cell plastids (chloroplasts)] that contain their own genomes, in autonomously maintained DNAs called plasmids, and in viruses. See also: Cell nucleus; Genetic code; Genetics; Genomics; Nucleic acid; Plasmid
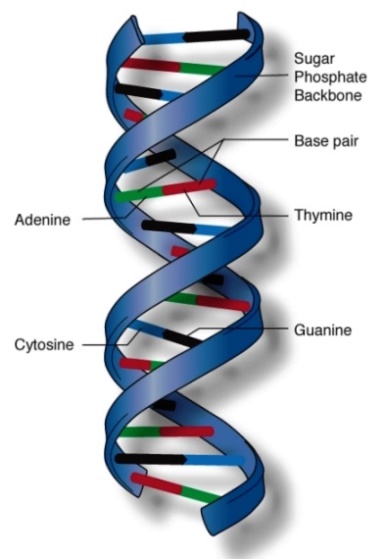
DNA is composed of two long polymer strands of the sugar 2-deoxyribose, phosphate, and purine and pyrimidine bases. The backbone of each strand is composed of alternating 2-deoxyribose and phosphate linked together through phosphodiester bonds (Fig. 2). A DNA strand has directionality: each phosphate is linked to the 3′ position of the preceding deoxyribose and to the 5′ position of the following deoxyribose. The four bases found in DNA are adenine (A), thymine (T), guanine (G), and cytosine (C). Each 2-deoxyribose is linked to one of four bases in the 1′ position via a covalent glycosidic bond. A base linked to a sugar is called a nucleoside, and a base linked to a sugar phosphate is called a nucleotide. The numbering of atom positions of the sugar is accompanied by a prime sign to distinguish it from the positional numbering of the bases. The sequence of these four bases allows DNA to carry genetic information. Bases can form hydrogen bonds with each other. Adenine forms two bonds with thymine, and cytosine forms three bonds with guanine. These two sets of base pairs have the same geometry, allowing DNA to maintain the same structure regardless of the specific sequence of base pairs (Fig. 2). See also: Nucleotide; Purine; Pyrimidine
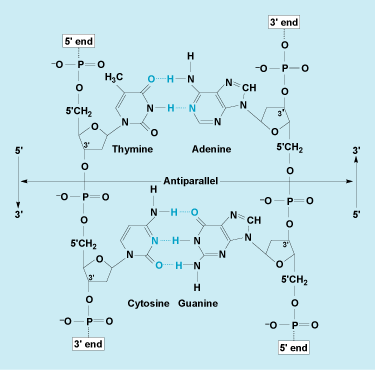
Structure
DNA can be single-stranded or aligned to form two-, three-, or even four-stranded structures. It is commonly found in its double-stranded form in chromosomes. In double-stranded DNA, the two strands wrap around each other to form a double helix (Fig. 3). The two strands are held together by base pairing and are antiparallel. Thus, if one strand is oriented in the 5′ to 3′ direction, the other strand will be 3′ to 5′. This double-helical structure of DNA was first proposed in 1953 by J. D. Watson and F. H. C. Crick. See also: Chromosome
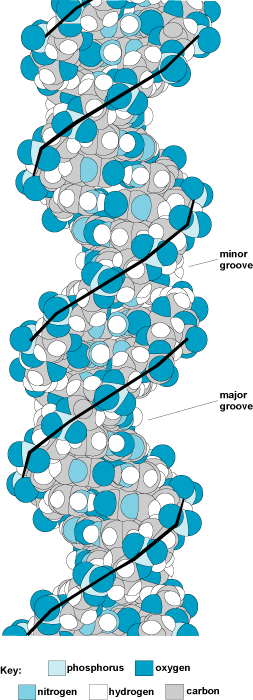
The most common form of DNA is the B-form, which is a right-handed double helix with 10.4 base pairs per turn (Fig. 3). In a B-form double helix, the ribose–phosphate backbones run along the outside of the helix, and the base pairs are roughly perpendicular to the axis of the helix and lie just offset from the center axis of the helix. This offset causes one of the two grooves that run along the helix to be larger than the other; thus, they are designated the major groove and the minor groove. Proteins that bind to DNA usually bind specifically to one of the two grooves. Less common forms of DNA include the A-form, which is a right-handed double helix that has 11 base pairs per turn and has a wider diameter than the B-form, and the Z-form, which is a narrow, irregular left-handed double helix (Fig. 4).
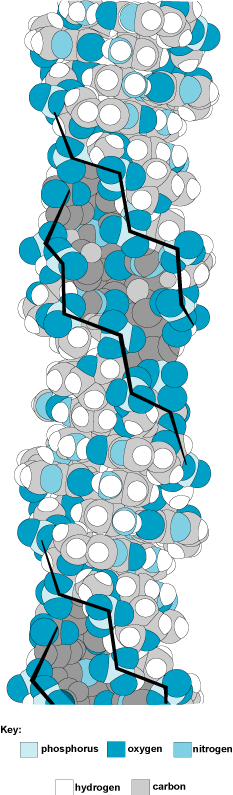
Right-handed conformation
Following Watson and Crick's proposal, experiments supported a right-handed form of the double helix in which there were approximately 10 base pairs per turn. In this form, each base pair is similar to the neighboring base pair, except that there is a rotation of approximately 36° between the base pairs. The conformation of DNA was determined by x-ray diffraction studies of fibers. A dried DNA fiber produces a type A pattern, in which the base pairs are tilted somewhat away from the helix axis. However, a wet or hydrated DNA fiber produces a B-type pattern, in which the bases are perpendicular to the helix axis (Fig. 3).
Left-handed conformation (Z-DNA)
In 1979, it was discovered that DNA can also form a left-handed double helix with the same hydrogen bonding between the bases. This was discovered by solving the structure of crystals of short DNA fragments; crystals can yield a more detailed view of the molecule. A solid line drawn between the phosphates in the left-handed conformation follows a zigzag path (Fig. 4); hence, it is called Z-DNA. In contrast to B-DNA, Z-DNA has only one deep helical groove extending almost to the axis of the helix. The base pairs in left-handed Z-DNA are located near the outside of the helix. B-DNA has a one-base-pair repeating unit, whereas Z-DNA has a dinucleotide repeating pattern. Both forms of DNA have the two sugar–phosphate backbones oriented in opposite directions (Fig. 2). Z-DNA has been identified in DNA fibers as well as inside living cells.
Conformational changes
In biological systems, DNA is normally subjected to a torsional strain that tends to unwind the double helix. This torsional strain tends to untwist right-handed B-DNA and sometimes is great enough to stabilize certain regions of DNA in the left-handed Z-DNA conformation. The conversion from right-handed B-DNA to left-handed Z-DNA is associated with changes in the relationship of the bases to the sugar backbone. Every other base in Z-DNA is rotated about the bond connecting it to the sugar. This altered form is common in adenine or guanine residues (purines), but less common in thymine or cytosine residues (pyrimidines); thus, sections that form Z-DNA often have alternations of purines and pyrimidines.
Although right-handed B-DNA is the most common form of DNA, left-handed Z-DNA occurs in a number of places and may play an important role in the regulation of gene expression. In addition, Z-DNA appears to be an important component of the process of crossing-over or recombination of DNA molecules. Z-DNA is important in carrying out special functions that are generally associated with special proteins that bind to Z-DNA and not to B-DNA. See also: Crossing-over (genetics); Gene; Recombination (genetics)
Function
For cells to live and grow, the genetic information in DNA must be (1) propagated and maintained from generation to generation and (2) expressed to synthesize the components of a cell. These two functions are carried out by the processes of DNA replication and transcription, respectively.
Replication
Each of the two strands of a DNA double helix contains all of the information necessary to make a new double-stranded molecule. During replication (Fig. 5), the two parental strands are separated, and each is used as a template for the synthesis of a new strand of DNA. Base incorporation is directed by the existing DNA strand; nucleotides that base-pair with the template are added to the nascent DNA strand The product of replication is two complete double-stranded helices, and each contains all of the genetic information (the identical base sequence) of the parental DNA. Each progeny double helix is composed of one parental and one nascent strand. DNA replication is very accurate. In bacteria, the mutation rate is about 1 error per 1000 bacteria per generation, or about 1 error in 109 base pairs replicated. This low error rate is the result of a combination of the high accuracy of the replication process and cellular pathways that repair misincorporated bases. See also: DNA repair; Mutation
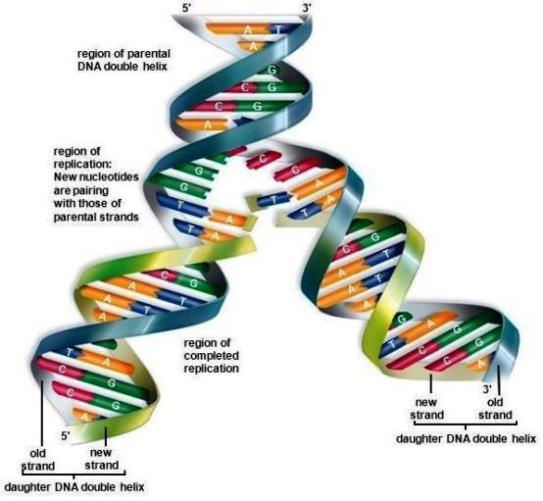
Synthesis of the nascent DNA strands is carried out by a family of enzymes called DNA polymerases. These enzymes synthesize a new phosphodiester bond between the 3′ hydroxyl position of the terminal deoxyribose of an existing DNA (or ribonucleic acid, RNA) chain and a deoxynucleotide triphosphate. All DNA polymerases synthesize new DNA in a 5′ to 3′ direction, require a primer (they must add on to the 3′ hydroxyl position of DNA or RNA), and require a DNA molecule as a template. DNA polymerases, in conjunction with multiple accessory proteins, can rapidly synthesize new DNA strands. (The mandatory participation of the 3′ hydroxyl forms the rationale for the use of nucleoside analogues that lack a 3′ hydroxyl as antiviral and anticancer drugs because they lead to premature chain termination.) A bacterial replication fork moves at a rate of approximately 1000 nucleotides per second; in eukaryotic cells, forks move at a rate of about 100 nucleotides per second (Fig. 6). See also: Enzyme
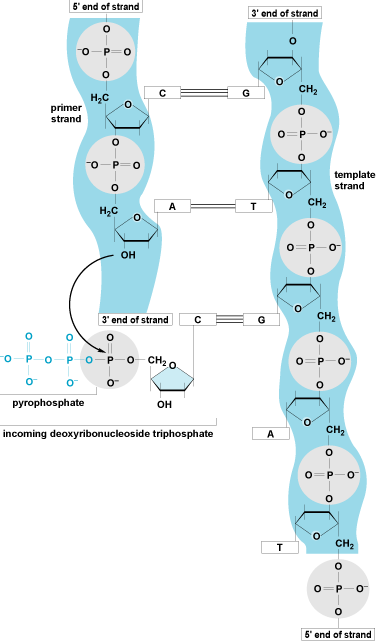
Transcription
In transcription, DNA acts as a template directing the synthesis of RNA. RNA is a single-stranded polymer similar to DNA, except that it contains the sugar ribose instead of 2-deoxyribose, and the base uracil (U) instead of thymine (Fig. 7). The two strands of DNA separate transiently, and one of the two single-stranded regions is used as a template to direct the synthesis of an RNA strand. As in DNA replication, base pairing between the incoming ribonucleotide and the template strand determines the sequence of bases incorporated into the nascent RNA. Thus, genetic information in the form of a specific sequence of bases is directly transferred from DNA to RNA in transcription. After the RNA is synthesized, the DNA reverts to a double-stranded form. Transcription is carried out by a family of enzymes called RNA polymerases. In prokaryotes, RNA polymerase interacts directly with the sites at which RNA transcription starts (promoters); in contrast, in eukaryotes, large complexes of proteins bind to promoters and then direct RNA polymerase to start RNA synthesis. Newly synthesized RNA is ultimately used to direct protein synthesis by ribosomes in a process called translation. See also: Protein; Ribonucleic acid (RNA); Ribosomes; Transcription
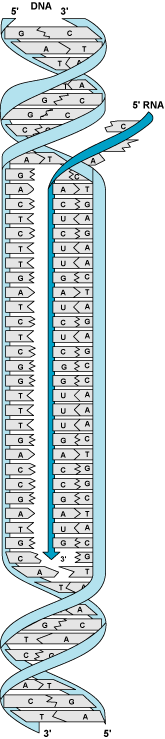
Genetic variation
There is a great deal of variation in the DNA content and sequences in different organisms. Because of base pairing, the ratios of adenine to thymine and cytosine to guanine are always the same. However, the ratio of adenine and thymine to guanine and cytosine in different organisms ranges from 1:4 to 3:4. There is also large variation in the amount of DNA in the genome of various organisms. The simplest viruses have genomes of only a few thousand base pairs, whereas complex eukaryotic organisms have genomes of billions of base pairs. (The lungfish has one of the largest genomes known, about 100 billion base pairs.) This variation partially reflects the increasing number of genes necessary to encode more complex organisms, but mainly reflects an increase in the amount of DNA that does not encode proteins. A large percentage of the DNA in multicellular eukaryotes is noncoding or is repetitive DNA (sequences that are repeated many times). This DNA can serve structural or regulatory functions, it can be related to transposable elements (DNA sequences that can insert into other unrelated sequences), or it has no known function. Rarely, genes are present in multiple copies to allow high levels of expression of certain cellular components. For example, most cells have hundreds or thousands of copies of the genes encoding the components needed for protein synthesis to ensure sufficient levels of expression of these components. See also: Transposable elements
In most eukaryotes, the DNA sequences that encode proteins are not continuous, but have other sequences interspersed within them. The sequences that are expressed in protein are called exons, and the interspersed sequences are called introns. The initial transcript synthesized by RNA polymerase contains both exons and introns and can be many times the length of the actual coding sequence. The RNA is then processed, and the introns are removed through a mechanism called RNA splicing to yield messenger RNA (mRNA), which is translated to make protein. See also: Exon; Intron
Recombinant technology
Techniques have been developed to allow DNA to be manipulated in the laboratory. These techniques, collectively called molecular biology or molecular genetics, have led to a revolution in biotechnology. This revolution began when methods were developed to cleave DNA at specific sequences and to join pieces of DNA together. Another major component of this technology is the ability to determine the sequence of the bases in DNA. There are two general approaches for determining DNA sequence: either chemical reactions are carried out that specifically cleave the sugar–phosphate bond at sites that contain a certain base, or DNA is synthesized in the presence of modified bases that cause termination of DNA synthesis after the incorporation of a certain base. A series of four reactions (one for each base) are carried out, and the lengths of the DNA fragments produced identify the sequence of the bases. These methods can now be automated so that it is practical to determine the DNA sequences of the entire genome of an organism. The complete sequences of humans and a number of experimentally important organisms have been determined. See also: Biotechnology; DNA sequencing; Genetic mapping; Human genome; Molecular biology
Another example of recombinant DNA technology is copy DNAs (cDNAs), which are made when an mRNA is converted to DNA using reverse transcriptase (a specialized DNA polymerase that can use RNA as a template). The resulting DNA can then be cloned and made in large quantity, and its sequence can be determined. Because it is much easier to manipulate DNA than RNA, cDNAs allow any RNA-based species to be characterized. See also: Genetic engineering; Reverse transcriptase
In the cell
The full genome of DNA must be substantially compacted to fit into a cell. For example, virtually every cell in the human body contains a full genome of DNA, which in turn has a total length of about 3 m (10 ft). However, this DNA must fit into a nucleus with a diameter of 10−5 m. This immense reduction in length is accomplished in eukaryotes via multiple levels of compaction in a nucleoprotein structure termed chromatin. To summarize, the first level involves spooling about 200 base pairs of DNA onto a complex of basic proteins called histones to form a nucleosome. Nucleosomes are connected like beads on a string to form a 10-nm-diameter fiber, and this is further coiled to form a 30-nm fiber. The 30-nm fibers are further coiled and organized into loops formed by periodic attachments to a protein scaffold. This scaffold organizes the complex into the shape of the metaphase chromosome seen during mitosis. See also: Histone; Mitosis; Nucleosome
The nucleosome is the fundamental structural unit of DNA in all eukaryotes. Virtually all of the DNA in each cell of an organism is packaged into nucleosomes. Nucleosomes are squat cylinders (6 nm × 11 nm × 11 nm) that are arranged as repeating subunits along the DNA at approximately 200-base-pair intervals. Pairs of four different histone proteins (H2A, H2B, H3, and H4) constitute an octamer complex, on the outside of which is wrapped 146 base pairs of DNA to form the nucleosome core. The DNA in the core is wrapped in 1.75 left-handed coils, resulting in approximately sixfold compaction of the DNA. The detailed structure of the nucleosome core (Fig. 8) has been determined by x-ray crystallography. Nucleosome cores are connected to one another by about 55 base pairs of linker DNA. The 146-base-pair length of DNA in the nucleosome core is invariant among all species studied, but the length of linker DNA can range from 20 to 100 base pairs among different species and different tissues. The reason for this variation is unknown. The linker DNA binds a fifth histone, H1, which plays a role in condensation of the 10-nm fiber into the higher-order structures.
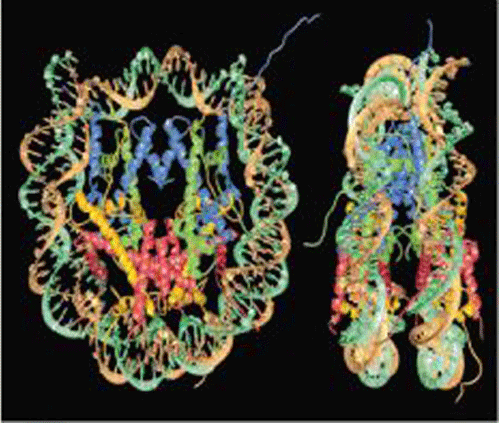
Nucleosomes reduce the accessibility of DNA to DNA-binding proteins such as polymerases and other protein factors essential for transcription and replication. One level of regulation of gene expression occurs via the posttranslational modification of histones. For example, specific protein factors activate transcription by adding acetyl groups to specific amino acid residues in the histones, whereas other factors with the opposite transcription effect remove those residues. Malfunctions in these regulatory processes have been associated with human diseases. There are several other types of histone posttranslational modifications with regulatory effects. Thus, chromatin structure not only compacts the genome, but also affords an additional level of control of gene expression. See also: Histone modifications, chromatin structure, and gene expression