The study of ancient climates. Climate is the long-term expression of weather; in the modern world, climate is most noticeably expressed in vegetation, soil types and characteristics of the land surface. To study ancient climates, paleoclimatologists must be familiar with various disciplines of geology, such as sedimentology and paleontology and with climate dynamics, which includes aspects of geography and of atmospheric and oceanic physics. A compelling need exists for societies to be able to predict climate change. Understanding the history of the Earth's climate system greatly enhances the ability to predict how it might behave in the future.
Information about ancient climates comes principally from three sources: sedimentary deposits, including ancient soils; the past distribution of plants and animals, as well as some morphological features such as the shapes of leaves or tree rings; and the chemical compositions of certain fossils and rock components. These are all known as proxy indicators of climate (as opposed to direct indicators, such as temperature, which cannot be measured for the past). In addition, paleoclimatologists use computer models of climate that have been modified for application to ancient conditions. See also: Climate history; Climatology; Geology; Geomorphology; Paleontology
Climate dynamics
Like modern climatologists, paleoclimatologists are concerned with boundary conditions, forcing and response. Boundary conditions are the limits within which the climate system operates. The boundary conditions considered by paleoclimatologists depend on the part of the Earth's history that is being studied. For the recent past, that is, the last few million years, boundary conditions that can change on short time scales are considered; for example, atmospheric chemistry. For the more distant past, paleoclimatologists must also consider boundary conditions that change on long time scales. Geographic features—that is, the positions of the continents, the location and orientation of major mountain ranges, the positions of shorelines and the presence or absence of epicontinental seaways (large areas of the continents flooded during times of high sea level)—are important for understanding paleoclimatic patterns. In addition, the solar constant and the rotation rate of the Earth have changed through geologic time and also must be considered in studying paleoclimates, particularly before about 4 × 108 years ago (shelly fossils first appeared in the record about 6 × 108 years ago). Forcing is a change in boundary conditions, such as continental drift and response is how forcing changes the climate system. Forcing and response are cause and effect in paleoclimate change. Paleoclimate change during the history of the Earth has taken place on time scales that are very short (hundreds of years) and very long (hundreds of millions of years). See also: Continental drift; Continents, evolution of; Paleogeography; Plate tectonics; Solar constant
Modeling ancient climates
The record of climate in sediments and rocks is remarkable because it illustrates a multitude of abrupt and slow changes in climate throughout Earth's history. The record is also incomplete. Many older rocks have been subject to erosion or metamorphism and farther back in time the record becomes increasingly sparse. In addition, paleoclimatologists do not have true “paleo” barometers or thermometers. Rather, paleoclimate is studied indirectly through its imprint on sediments and organisms. Thus, ancient climates cannot be reconstructed completely on a global scale. However, the available data are useful if climate is modeled; the data can then be used to test aspects of the model predictions and thereby the model itself.
Although many aspects of paleoclimate change are actively studied, some topics are particularly important in that the studies are challenging traditionally accepted ideas. These topics include the role of mountain ranges in determining global climate patterns, the climate systems of supercontinents, warm polar climates, the role of ocean circulation in global climate and the role of carbon dioxide in long-term climate change.
The study of paleoclimates has benefited greatly from the application of comprehensive computer models. The development of atmosphere and ocean models based on fundamental physical laws of fluid motion, energy and momentum was at the leading edge of research in the atmospheric and oceanic sciences during the 1980s. Very rapid development in predictive model capability has occurred because of increased supercomputer power, advances in programming and a growing inventory of observations about the modern climate system. See also: Climate modeling
Paleoclimate indicators
Proxy indicators of paleoclimate are abundant in the geologic record. Many were first discovered during the nineteenth century and helped scientists and naturalists appreciate the dynamism of the Earth's history. Fossil palm leaves and crocodiles in the Canadian Arctic Islands and thick coal seams in Antarctica are among the many seemingly anomalous occurrences that indicate that climate has changed. Many of the changes are explained by continental drift, but other changes represent fundamental differences between modern climate patterns and ancient ones.
Sedimentary indicators
Important sedimentary indicators forming on land are coal, eolian sandstone (ancient sand dunes), evaporite (salt), tillite (ancient glacial deposits) and paleosols. Coal may form where conditions were favorable for growth of plants and accumulation and preservation of peat, conditions that are partly controlled by climate, especially seasonality of rainfall. Eolian sandstone and evaporite are indicative of arid climates. Paleosols record water-table levels and intensity of weathering, both of which are dependent on temperature or rainfall. Paleosols also provide important information about the type of vegetation (see next section). Certain marine sediments also provide important information about ancient atmospheric and oceanic circulation patterns. These are bedded chert, which is formed from the siliceous shells of microscopic marine plants and animals and phosphate, both of which form in upwelling zones, that is, zones of high biologic productivity in the oceans. At present, these zones owe their distribution to global wind patterns and thus geologic evidence of upwelling helps in the interpretation of ancient atmospheric circulation. Extensive deposits of sediments rich in organic substances also provide information about productivity in the oceans. In addition to specific types of sedimentary rock, patterns of sedimentation also provide information about paleoclimates. Layering in lake deposits, for example, may be controlled by seasonal changes that are, in turn, controlled by climate. Much of the understanding of more recent climate change has come from such deposits. See also: Chert; Coal; Depositional systems and environments; Laterite; Marine geology; Marine sediments; Paleosol; Saline evaporites; Sedimentology; Stratigraphy; Upwelling
Fossil indicators
Fossils provide information about climate mostly by their distribution (paleobiogeography), although several specific types of fossils may be indicative of certain climatic conditions. Paleobiogeographic patterns commonly follow climatic gradients such as temperature and rainfall; they are useful qualitatively and sometimes quantitatively, for delineating those gradients.
In addition to indicator species and paleobiogeographic patterns, fossils, particularly plants, commonly show morphological features that are related to climate. The morphology of leaves of woody angiosperms (flowering plants) is controlled by mean annual temperature, mean annual range of temperature, mean annual precipitation and seasonality (Fig. 1). The thickness and structure of the cuticle (waxy outer layer) of angiosperm and other types of leaves are partly dependent on water supply. In addition, the cuticle contains a record of the type and density of stomata (pores through which gas is exchanged between the atmosphere and the leaf interior). Stomata have different forms depending on the aridity of the climate, but more important, the density of stomata in some plants is sensitive to the amount of CO2 in the atmosphere. Leaf-margin analysis of angiosperms has provided a quantitative tool for the estimation of several climate parameters. For example, the proportion of smooth-margined versus toothed-margined leaves is highly correlated with the mean annual temperature. Growth rings in fossil wood are sensitive to frosts, rainfall and temperature during the growing season. See also: Biogeography; Dendrochronology; Fossil; Paleobotany
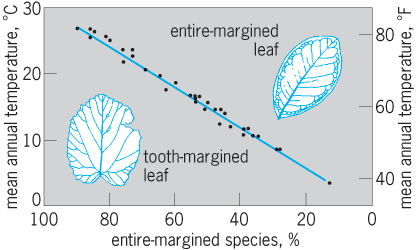
Geochemical indicators
The third type of information available for documenting paleoclimate patterns and change is stable-isotope geochemistry of fossils and certain types of sedimentary rock, as well as other geochemical parameters such as elements ratios. Many elements that are used by organisms to make shells, teeth and stems occur naturally in several different forms, known as isotopes. The most climatically useful isotopes are those of oxygen (O) and carbon (C). Most oxygen occurs as 16O, an atom with eight protons and eight neutrons in the nucleus; however, some oxygen occurs as 18O, which has two extra neutrons. The rate at which the two isotopes are cycled through the oceans and atmosphere and taken up by organisms differs in response to a number of factors, including temperature; the biology of the organisms also has a strong effect. In the oceans, organisms living in tropical waters have a higher proportion of 16O in their shells compared with those living in polar waters. In addition, the proportions of the two isotopes available in the oceans depends on the amount of ice on the Earth's surface. Although the effects of temperature change and ice-volume change can be difficult to distinguish, the analysis of oxygen isotopes in marine fossils has provided a powerful quantitative tool for the study of both long-term temperature change and the history of the polar ice caps.
On the continents, the ratios of 18O to 16O in fossils and rocks are controlled primarily by the original isotopic composition of the water, precipitation and or evaporation, which can vary widely in different areas. Mapping the variations can allow analysis of the movement of air masses, the type of precipitation (for example, waters derived from snowmelt are generally much richer in 16O than those derived from rain) and so on. Changes in the isotopic ratios through time can reveal patterns of seasonality as well a longer-term climate change.
The isotopes of carbon are 12C and 13C (14C, which is radioactive and is used in dating, is not used for climate studies). Organic matter (the soft tissues of organisms) is rich in 12C compared with most other carbon-containing materials. Changes in the proportions of 12C and 13C, especially in marine fossils and rocks, can be indicative of changes in biologic productivity and burial of organic matter (large-scale burial of organic matter sequesters 12C in rock). Because the carbon isotopic composition of the atmosphere and plant roots is so different, the composition of paleosol nodules, especially calcite nodules (caliche), can reflect the amount of CO2 in the atmosphere. See also: Caliche; Geologic thermometry; Isotope
Causes of paleoclimate change
The rarest of paleoclimate changes is that which can be attributed confidently to a single cause. A great deal of research in paleoclimatology has been devoted to understanding the causes of climatic change and the overriding conclusion is that any given shift in the paleoclimate history of the Earth was brought about by multiple factors operating in concert. Nevertheless, some forcing factors have been identified as being particularly important. The most important forcing factors for paleoclimatic variation are changes in paleogeography and atmospheric chemistry and variations in the Earth's orbital parameters.
Paleogeographic forcing operates on relatively long time scales, millions to tens of millions of years and occurs in a variety of modes. Changes in continental positions may close off or open up new routes for ocean currents, which can change the distribution of temperature over the Earth's surface. For example, the opening of the Drake Passage between South America and Antarctica is regarded as a contributing factor to the formation of the Antarctic ice cap. While land connection between the two continents existed, the circumpolar current was deflected equatorward along the western side of the coast, while relatively warm water was deflected poleward along the eastern side. With the opening of the Drake Passage, however, the circumpolar current became continuous, isolating Antarctica from the warm, low-latitude waters. Although the paleogeographic change that brought about this isolation—the drifting of Antarctica and South America away from each other—occurred slowly, the climatic change that resulted was relatively rapid. This is an example of a paleoclimate threshold; the climate system seems to resist change until finally the forcing becomes too great and then the adjustment takes place quickly.
Sea-level changes also may have brought about paleoclimate changes. Sea level is partly controlled by changes in ice volume at the poles and thus may sometimes be an effect, rather than a cause, of climate change. However, sea level also changes in response to mountain-building activity and continental drift and these changes may be forcing factors for paleoclimate change. In general, times of high sea level were times of warmth. However, this pattern is not necessarily true in detail, indicating that other factors also must play a role. Among these factors are the location, size and orientation of mountains and epicontinental seaways.
Most paleoclimatologic research on atmospheric chemistry has been directed toward modeling the variations in carbon dioxide content of the atmosphere. Carbon dioxide is well known as a greenhouse gas that traps heat at the Earth's surface. Carbon dioxide content has varied in the past and strong evidence for such variations during the recent past (the last few thousand years) has been gathered by using detailed records of atmospheric bubbles in ice cores and stable isotopes (Fig. 2). In addition, the carbon cycle is relatively well known and changes in carbon dioxide content in the atmosphere must have taken place, because large fluctuations are observed in the fossil record for other reservoirs for carbon. Finally, carbon dioxide changes appear to be the only mechanism that can account for some global temperature changes. However, the effect is not necessarily linear. Some times of global warming were times of lower carbon dioxide in the atmosphere, thus illustrating the complexity of the climate system. Another important greenhouse gas is methane, which has a much stronger greenhouse effect than carbon dioxide. Methane is very enriched in 12C and there are numerous records throughout geologic time of sudden increases in that isotope in rocks and fossils. These sudden increases are sometimes proposed as evidence for sudden releases of large amounts of methane from ocean sediments or swamps. Such releases might be expected to have a strong perturbing effect on climate. See also: Atmospheric chemistry; Biogeochemistry; Carbon dioxide; Greenhouse effect; Methane
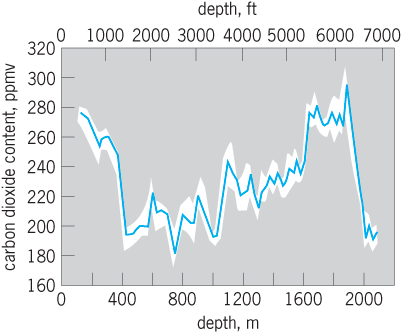
Characteristics of the Earth's orbit around the Sun vary regularly and lead to cyclical variations in the amount and distribution of solar radiation reaching the Earth. The three characteristics that are most important to paleoclimate studies are precession, obliquity and eccentricity. Precession of the equinoxes determines the time of year when the Earth is closest to the Sun; in the present geological era, this occurs in January. Precession varies with a period of about 19,000–23,000 years. Obliquity is the tilt of the Earth's axis relative to the plane of the Earth's orbit around the Sun; at present, obliquity is about 23.5°. Obliquity varies with a period of about 41,000 years. Eccentricity is the shape of the Earth's orbit around the Sun, which varies from circular, allowing solar input to be constant throughout the year, to oval, which creates strong annual variability. Eccentricity varies with a period of about 100,000 years. These are known as Milankovitch cycles, after the Yugoslavian astronomer who developed mathematical models of the cycles. Because the different cycles possess different periods, the paleoclimatic response to Milankovitch cyclicity has been complicated. Nevertheless, Milankovitch cyclicity has been described from sedimentary rocks of many parts of Earth history. See also: Earth rotation and orbital motion; Precession of equinoxes
Paleoclimate history
The paleoclimate history of the Earth can be divided into several stages, based on events and on the increasing resolution in the geologic record toward the present. See also: Geologic time scale
Pre-Phanerozoic climate
Paleoclimate history before the appearance of the first shelly fossils is poorly known because many of the strongest types of evidence for paleoclimate studies do not exist in pre-Phanerozoic rocks. It is clear, however, that the interval was punctuated by extensive glaciations, as indicated by the abundance of tillites.
Pre-Pangaean Paleozoic
During the early part of the Phanerozoic, land consisted of Gondwana, which would later break up into the modern continents of the Southern Hemisphere and pieces of southern Asia and numerous small continents, which were to become the modern Northern Hemisphere continents. Sea level was high and temperatures were generally warm through much of the interval, although sea level and temperatures were low at the very beginning of the interval and large ice sheets formed near the South Pole at the end of the Ordovician Period and again during the early Carboniferous. Reefs were widespread during the Ordovician through Devonian and peat swamps were widespread during the Carboniferous. See also: Bog; Carboniferous; Devonian; Ordovician; Peat; Reef; Phanerozoic
Pangaean climate
By the end of the Paleozoic, nearly all the continents had come together to form the supercontinent, Pangaea. At the same time, global climate changed dramatically. Abundant evidence exists for aridity or strongly seasonal rainfall. These changes were brought about by the influence that the large landmass had on atmospheric circulation, creating an intensely seasonal climate. Sea level was very low at this time, increasing the size of the exposed continent and augmenting its climatic influence. Temperature is perhaps most difficult to determine for this time because biogeographic patterns, so useful in the previous interval, are extremely weak and oxygen isotopic determinations of paleotemperatures are still unreliable. See also: Paleozoic
Late Mesozoic to Early Tertiary climate
With the breakup of Pangaea, sea level rose and climate again changed, becoming wetter in many parts of the globe and probably cooler. Leaf-margin analysis is possible from this time forward, because flowering plants had evolved and dispersed across the globe; and relatively reliable and abundant oxygen-isotope analyses also begin to be possible for about the same time. The Middle Cretaceous through Early Tertiary was warm relative to later paleoclimates, but it was punctuated by cooling events. In addition, coal, phosphate and marine rocks rich in organic substances were abundant at various times during this interval. Particularly impressive was a short-lived but extremely intense episode of deposition of organic-rich rock worldwide. See also: Cretaceous; Mesozoic; Tertiary
Late Tertiary
Climate cooled substantially in several steps through the latter part of the Tertiary Period (Fig. 3). Ice caps at both poles were formed, first in the Southern Hemisphere. Rapid and significant changes in oceanic biogeographic patterns took place and the foundations of the modern vegetation patterns were established.
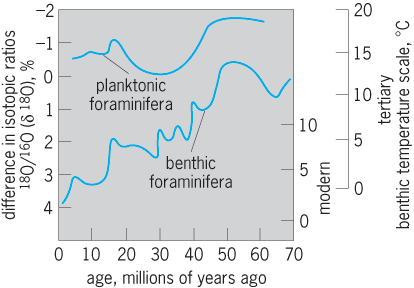
Quaternary
The Quaternary Period includes the major Northern Hemisphere glaciations of the last 2 × 106 years. Although there is no reason to regard these as different in scale from earlier glacial epochs in the Earth's history, the proximity of these events to the present day has permitted paleoclimatologists to study them in far greater detail than is possible for the earlier events. Much of the understanding of the dynamics of continental glaciations and paleoclimate has come from studying the global patterns of the Quaternary. See also: Glacial history; Quaternary
Practical applications of paleoclimate research
Understanding paleoclimate is important for two human concerns: exploration for resources and predicting future climate change. The distribution of many energy and industrial resources (such as coal, petroleum, phosphate, manganese and so on) is at least partly related to the climate of the times in which they were formed. Knowing past climate patterns aids in the exploration for new resources. The Earth's climate system has undergone many extreme changes, some far beyond those predicted for future anthropogenic climate change. There is some evidence that the models we are using to predict future climate change, which are based on our understanding of climate in the present and recent past (the last 10,000 or 2 million years), may not be sufficient to understand these more extreme states. By studying the full range of climate change in the Earth's history, we gain a better understanding of how climate will respond to possible forcings. See also: Climatic prediction; Geophysical exploration