Key Concepts
The large-scale, systematic, and irreversible changes over time of the structure and composition of a star over time. All stars have "lives" in the sense that they are born, age, and die. A stellar "death," or end stage of evolution, ranges from an eons-long smolder as a white dwarf for typical stars, to explosive self-destruction as a supernova for massive stars. The initial mass of a star is the overwhelmingly determinative property of the evolutionary path that the star will follow (Fig. 1). See also: Star
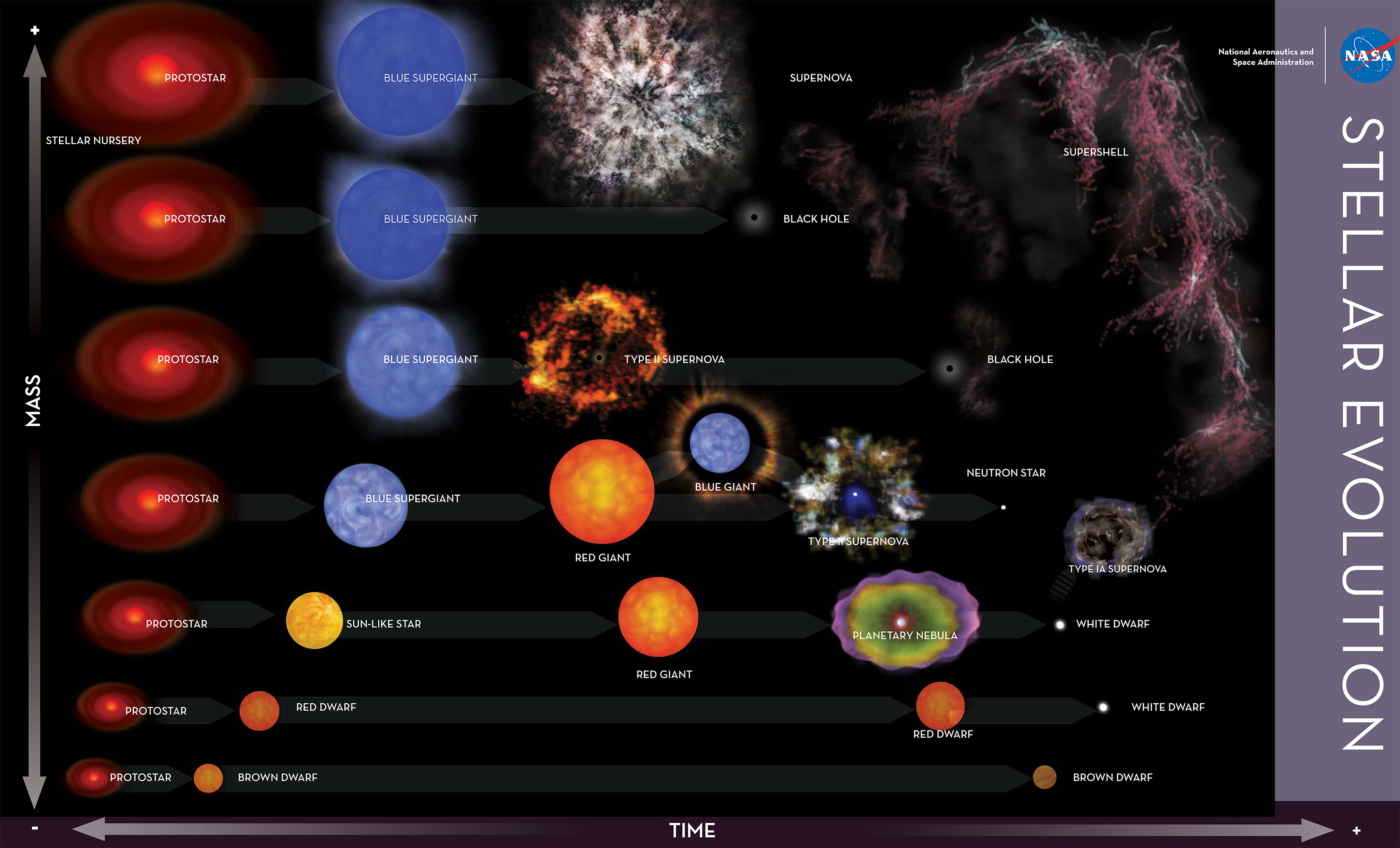
Types of stars
Dozens of different types of stars populate the Milky Way Galaxy and the universe at large (Fig. 2). The most common are main-sequence dwarfs like the Sun that fuse hydrogen into helium within their cores. Dwarfs run the full gamut of stellar masses, from perhaps as much as 200 solar masses (200 M) down to the minimum of 0.075 solar mass, beneath which the full fusion proton-proton chain does not operate. Main sequence dwarfs occupy a spectral sequence from class O through classes B, A, F, G, K, M, and L. Within the main sequence, dwarf stars break into two broad groups: those under 1.3 solar masses (class F5), whose luminosities derive from the proton-proton chain, and higher-mass stars that are supported principally by the carbon cycle. Among main sequence stars, the most common are M stars, also known as red dwarf stars, representing around 80% of stars in the Milky Way. Below the end of the main sequence (masses less than 0.075 M
) lie the brown dwarfs that occupy half of class L and all of classes T and Y. Brown dwarfs shine both from gravitational energy and from fusion of their natural deuterium. See also: Brown dwarf; Carbon-nitrogen-oxygen cycles (astrophysics); Deuterium; Nuclear fusion; Proton-proton chain; Spectral type
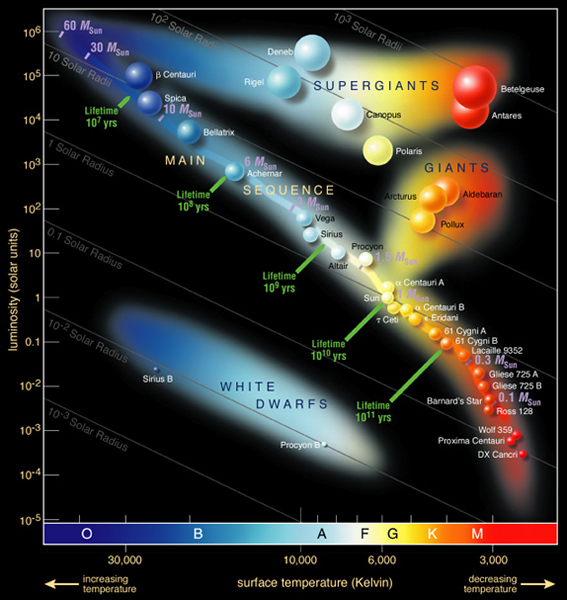
Scattered among the dwarfs are different kinds of stars that are distinguishable through comparison with the main sequence dwarfs by their sizes and luminosities: luminous giants (with maximum radii equaling that of the inner solar system), brilliant supergiants (maximum radii comparable to the orbits of Jupiter and Saturn), subgiants (which fall between giants and dwarfs), and dim white dwarfs (the size of Earth). All these classifications may be broken into subclasses. The list also includes the central stars of planetary nebulae, double stars, novae, supernovae, neutron stars, and black holes. The study of stellar evolution seeks to determine where they all came from. Do they stand alone, or are they somehow connected? If the latter, what kinds of stars precede and succeed what other kinds?
Stellar models
Stars age so slowly that it is impossible—except under rare circumstances—to see the transformations take place. Instead, stellar theorists, using the laws of physics, build numerical models of stars and then age them. The procedure is simple in principle, but difficult in practice. At the start, a star is in hydrostatic equilibrium, in which each layer supports the layers above it, with the pressure difference across a layer equaling the weight of the layer, and is internally supported by thermonuclear fusion. The relation between pressure, density, and temperature is given by the perfect gas law, P = NkT, where P is pressure, N is the number density of atomic particles, k is Boltzmann's constant, and T is temperature. Other equations involve the mass within a given radius, the rate at which energy is generated at a given radius, and the temperature gradient, which controls convection. As fusion proceeds and the rate of energy generation and the internal chemical composition change, the structure of the star changes as well. Stepwise calculations that predict luminosity, radius, and effective temperature then allow the star to be followed as it “moves” (changes in effective temperature and luminosity) on the Hertzsprung-Russell (HR) diagram. When appropriate mass loss is taken into account, most of the different kinds of stars fall into place. See also: Gas; Hertzsprung-Russell diagram; Hydrostatics; Thermodynamic processes
Star formation
Stars are born from compact knots of gas and dust within molecular clouds (Fig. 3). If dense enough, the knots start to contract under their own gravity. Conservation of angular momentum demands that as the knots collapse, they must spin faster. Magnetic fields transport some of this angular momentum away, and over time, a flattened disk of material—known as a protoplanetary disk—forms around the collapsing, budding star, which at this point in its evolution is known as a protostar. The protoplanetary disk contains the materials from which planets later accumulate. See also: Angular momentum; Molecular cloud; Planet; Protostar
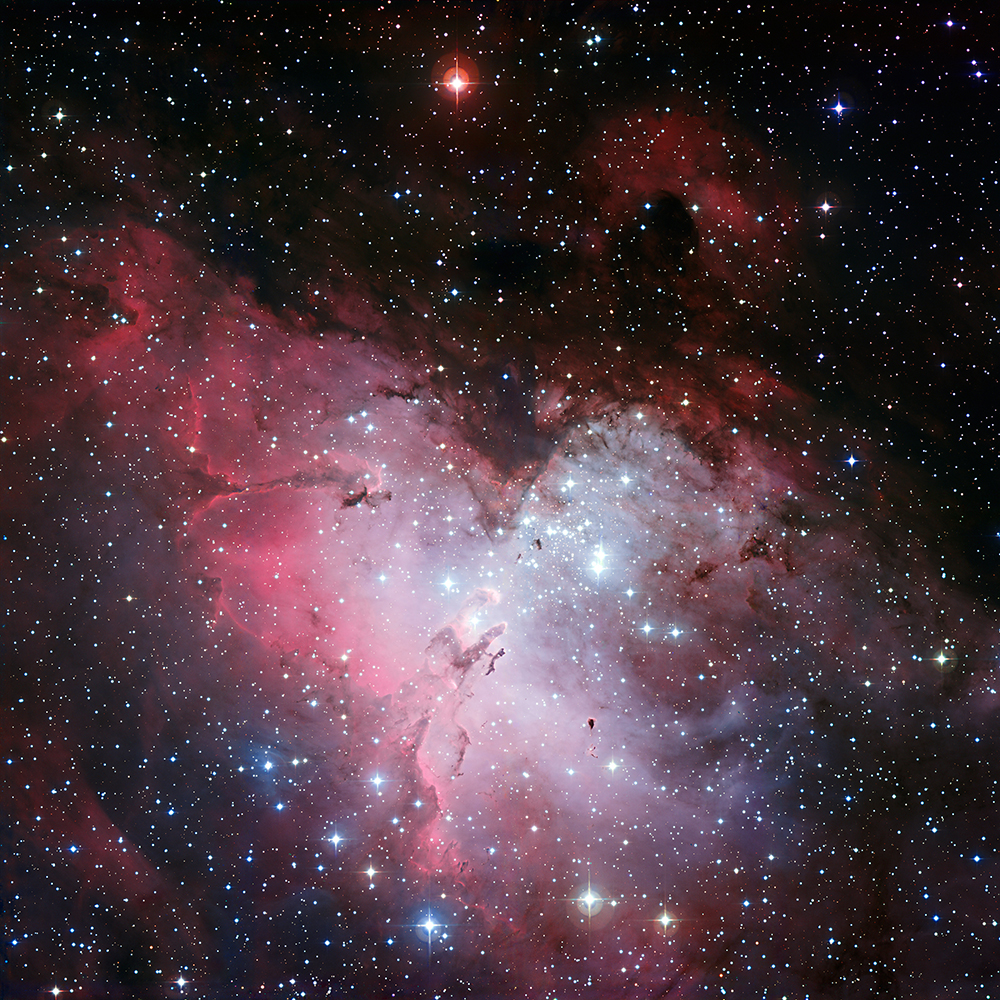
When the protostar's interior reaches about 1 million K (1 million °C or 1.8 million °F), it can fuse its internal deuterium. That and heat convection, which brings in fresh deuterium from outside the nuclear-burning zone, bring some stability, and a star can now be said to have been born. Stars like the Sun shrink at constant temperature until deuterium fusion dies down. Then the young stars heat at roughly constant luminosity until the full proton-proton chain begins, which provides the stars' luminosity and support and stops the gravitational contraction. The stars then settle onto the zero-age main sequence from which they will later evolve. At the same time, the surrounding dusty cloud is clearing, allowing new, accreting, and highly active so-called T Tauri stars to be seen flocking around their birth clouds (Fig. 4). The whole process takes only 10 or so million years, with the mature stars eventually leaving their birthplaces as they orbit within their host galaxy. High-mass stars proceed similarly, but at such a great pace that the death process begins even as the birth process is ending. See also: Heat convection; T Tauri star
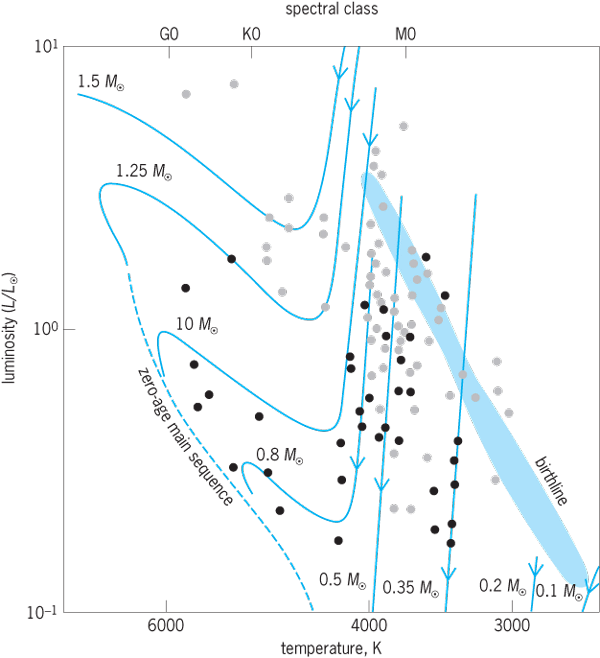
Early evolution
The main sequence is that zone on the HR diagram in which stars are stabilized against gravitational contraction by fusion. The higher the stellar mass, the greater the internal compression and temperature, and the more luminous the star. Hydrogen fusion is highly sensitive to temperature, with a small increase in stellar mass meaning a much higher fusion rate. Although greater mass means a greater nuclear-burning core mass and therefore a larger fuel supply, the increased fusion rate more than offsets this and thereby shortens stellar life. A star's fuel supply is proportional to its mass (M), while the rate of fusion is expressible by the luminosity (L). The average mass-luminosity relation found from binary stars is L ∝ M3.5. If a star's lifetime t is proportional to (fuel supply)/(rate of use), then t ∝ M / L ∝ M / M3.5 = 1 / M2.5. While the new Sun was destined to survive on the main sequence for 10 billion years, a 0.1-solar-mass star will live there for 10 trillion years, while a 100-solar-mass star will exhaust its core hydrogen in only 2.5 million years.
Main-sequence life, while stable, is not altogether quiet. Even there, stars change and evolve. As hydrogen “burns” to helium, four particles (protons) are converted to one (a helium nucleus). The pressure of a gas depends on the number of particles per unit volume, not on their kind. The result is a slow shrinkage of the core, which increases the temperature, raises the fusion rate, and causes the core to eat into the surrounding hydrogen envelope, incorporating fresh fuel. As a result, main-sequence dwarfs slowly brighten and eventually expand and cool some at their surfaces. (The Sun will more than double in brightness, dooming life on Earth, long before its core hydrogen is gone.) As a result, the main sequence spreads itself into a band toward the cool side (to the right) of the zero-age main sequence in an HR diagram, with the band widening toward the top.
Clusters of stars are born together in both space and time, most of them (presumably) with fully intact main sequences. As a cluster ages, stars peel off the main sequence to become giants and supergiants from the top down. A star cluster can be dated by where its main sequence ends. Open clusters, which occupy galactic disks, range from just-forming to about 10 billion years old, which gives the disk's age. Globular clusters, however, and for instance those in the Milky Way Galaxy's halo, have burned their main sequences down to around 0.8 solar. This process takes roughly 12 billion years, which is close to the age of the Galaxy. See also: Globular clusters; Milky Way Galaxy; Star clusters
The main sequence is divided into three parts. Below 0.8 or 0.9 solar mass (roughly class G8), no star has ever had time to evolve. Low-mass (K and M dwarf) evolution is therefore of only academic interest. Stellar evolution deals with less than 15% of the stars. Their fates depend again on mass. Between 0.8 solar mass and around 9 solar masses (classes G8 to B1), the stars die as white dwarfs. Above this range (classes O and B0), they explode. Binary, trinary, and even higher numbers of closely associated stars in a shared star system contribute further to the richness of stellar phenomena.
Intermediate-mass evolution
Main-sequence life lasts until the core hydrogen is almost gone, at which time hydrogen fusion shuts down rather suddenly. With no support, the now-quiet helium core can contract more rapidly under gravity's force. It heats, causing hydrogen fusion to spread into a thick, enclosing shell that runs on the carbon cycle. With a new (though temporary) energy source, the star first dims somewhat while it expands and cools at the surface, changing its spectrum to class K. The transition takes only a few hundred million years or less, leaving few stars in the middle of the HR diagram, with those stars with lower masses appearing as F, G, and K subgiants.
The consequences again depend on mass. As core contraction proceeds beyond the rightward transition in the HR diagram, stars from about 1 to 5 solar masses (still fusing hydrogen in a shell) suddenly and dramatically increase their luminosities. The future Sun will eventually grow 1000 times brighter than it is today, and a 5-solar-mass star (which begins at about 600 solar luminosities) will reach nearly 3000 solar. At the same time, the stars swell to become red giants. While the core (roughly half a solar mass) of the future Sun shrinks to the size of Earth, the radius will expand to that of Mercury's orbit, or even beyond. When the core temperature climbs to 100 million K, helium nuclei (alpha particles) begin fusing to unstable beryllium (8Be), which quickly decays back to alpha particles, setting up an equilibrium. The tiny amount of 8Be present reacts with additional alpha particles to create carbon via the triple alpha process:


(The “skip” over lithium, beryllium, and boron renders these elements rare.) The initiation of the triple-alpha process is explosive in stars like the Sun, because the core electrons become degenerate, like those that support white dwarfs. (The sudden burst of energy is absorbed and does not reach the stellar surface.) Fusion with additional helium nuclei creates oxygen and even neon. Above 2 solar masses, helium burning starts more quietly (Fig. 5). See also: Nucleosynthesis
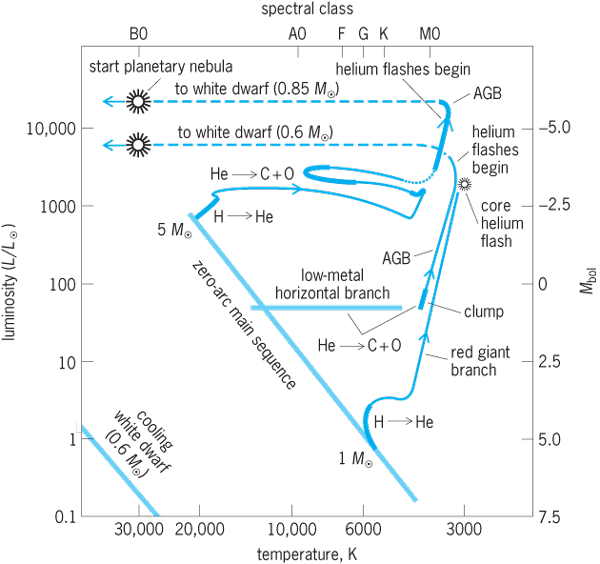
The star, now stabilized by a helium-burning core that is surrounded by a hydrogen-fusing shell, retreats about halfway down the red giant branch. The numerous lower-mass stars reside in the class K “red giant clump.” Low-mass metal-deficient globular cluster stars that have suffered different rates of mass loss spread out from the clump toward higher temperatures to create the distinctive horizontal branch. Energy-generating fusion reactions will try to proceed toward iron (the most stable of all nuclei). Some 80% of the energy is generated in hydrogen burning, so (discounting other burning modes) the helium-burning stage lasts only around 20% of the main-sequence lifetime. From 5 solar masses up, evolution proceeds similarly, but instead of settling into a distinct location on the HR diagram, the stars loop to the blue (higher temperatures), where they fuse helium as class G, F, and A giants. This change in evolutionary style is not abrupt, but gradual with increasing mass. See also: Giant star
Asymptotic giant branch (AGB)
When the helium has fused to carbon and oxygen, the core again contracts. Helium fusion spreads outward into a shell, and for the second time the star climbs the HR diagram's giant branch. Because the second climb is roughly asymptotic to the first, the second climb creates the asymptotic giant branch. The shrinking carbon-oxygen core is now surrounded by a shell of fusing helium, while the old hydrogen-fusing shell expands, cools, and shuts down. Eventually, however, the helium shell runs out of fuel, and the hydrogen shell reignites. Hydrogen burning feeds fresh helium into the space between it and the carbon core, and when there is enough of it, helium burning reignites explosively in a helium flash (or thermal pulse) that can affect the star's surface. The flash squelches hydrogen fusion, and the whole process starts again, with helium flashes coming at progressively shorter intervals. AGB stars become larger and brighter than before, passing into the cool end of class M, where they eventually become unstable enough to pulsate as long-period variables (Miras). The Sun will become 5000 times brighter than now and will reach out to the Earth's orbit, perhaps destroying the planet. More massive Miras can exceed the size of the Martian orbit. See also: Mira; Variable star
During this activity, stars go through various stages of convective dredge-up, in which they can raise elements created in their nuclear-burning zones to the stellar surfaces. The first of these dredge-ups alters the nitrogen and carbon isotope ratios while the star is on its first ascent of the red giant branch. Above about 3 solar masses, a second dredge-up in the early AGB stage can increase both surface nitrogen and helium. In the helium-flashing state, even carbon from helium fusion can be brought upward. Moreover, a host of elements created by slow neutron capture are elevated as well, including zirconium, strontium, barium, and many others. When the carbon abundance equals that of oxygen, an S-type star is seen (loaded with fresh zirconium); when carbon exceeds oxygen, a genuine carbon star is seen. See also: Carbon star
Mass loss and planetary nebulae
During the giant stages, stellar winds greatly increase. Mira pulsations cause shock waves that help drive mass from the stellar surfaces, where the cooled gas becomes ever richer in molecules, some even condensing into dust grains. High luminosity pushes the dust outward, and the dust couples with the gas, resulting in slow (10 km/s or 6 mi/s), thick winds that are tens of millions of times stronger than the solar wind (up to 10−5 solar mass per year). Because stars are in this state for hundreds of thousands of years, they will lose much of themselves back into space—the Sun nearly half of its mass, a 9-solar-mass star more than 80%. Advanced giants and Miras lose so much that they can visually disappear within dust clouds. Carbon star winds contain various kinds of carbon dust as well as organic molecules, while oxygen-rich stars produce silicate dust. The dust blown into interstellar space helps make new generations of stars. The elements created in the nuclear-burning shells are added to the interstellar inventory, where they too find their way into new generations of both stars and planets. A good portion of the carbon, most of the nitrogen, and significant fractions of the other elements come from such winds.
So much mass is lost that an evolving star becomes stripped nearly to its fusion zone, which is protected from the outside by a low-mass hydrogen envelope. As the inner region becomes exposed, the wind diminishes in mass but increases in speed and temperature. Hammering at the surrounding dusty, molecule-filled shroud of lost mass, the high-speed wind compresses the inner edge into a thick ring. Eaten away from the top by the wind and from below by fusion, the stellar envelope shrinks, slowly exposing the hot shell-core structure beneath. When the stripped star's surface reaches 25,000 K (24,700°C or 45,000°F), the dense ring that its high-speed wind had previously created is slowly ionized, with the degree being dependent on stellar temperature. Subsequent recapture of electrons by ions, along with collisional excitation of heavy atoms, causes the shell to glow, and a planetary nebula is born (Fig. 6). The first planetary nebula was announced in 1785 by German-born English astronomer William Herschel, who named the object class on the basis of their disklike shapes similar to planets when viewed in telescopes of the time. See also: Planetary nebula
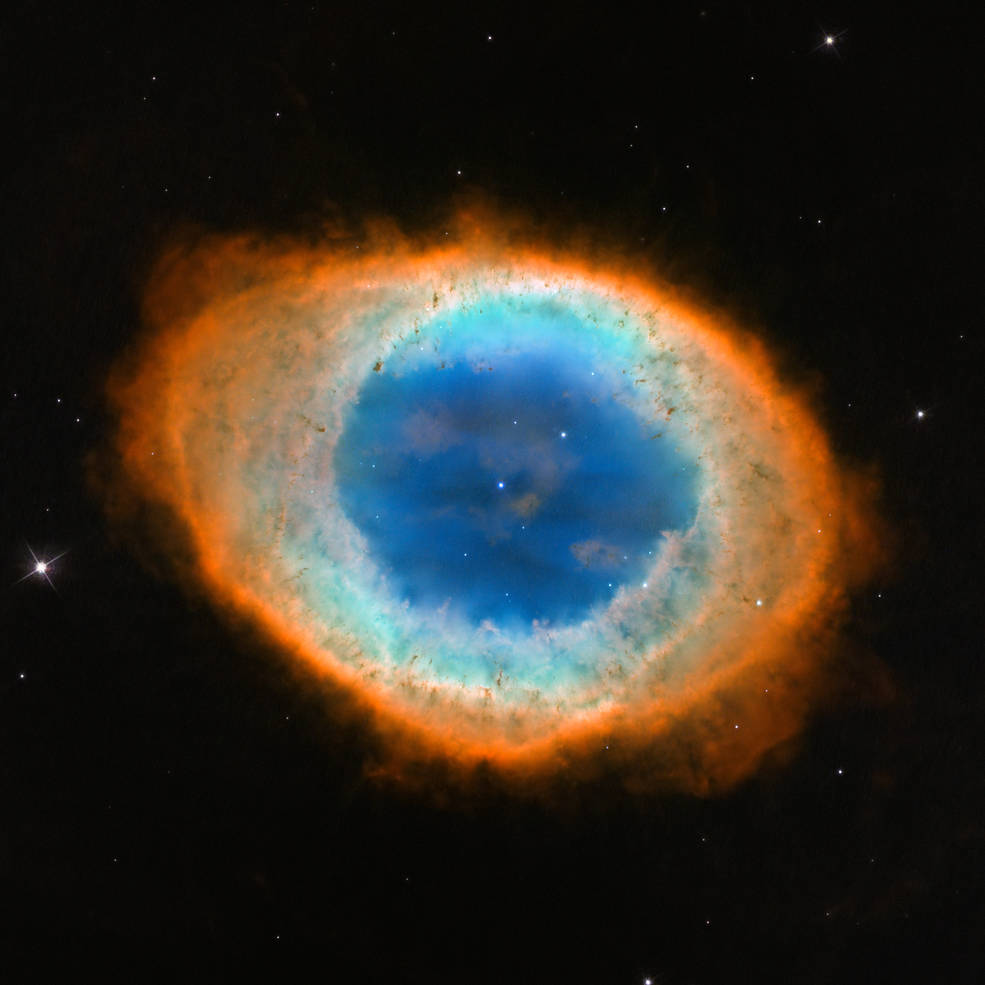
As the planetary nebula, expanding at about 20 km/s (12 mi/s), grows in size, it eventually becomes so tenuous that the ionizing radiation bursts through, allowing much of the full structure to be seen. After some 50,000 years, the nebula invisibly merges with the interstellar medium. During this time, the star inside first heats at constant luminosity to over 100,000 K (99,700°C or 180,000°F), with the luminosity and final temperature depending on the old core's mass (which ranges from around 0.5 M to nearly 1.4 M
, the Chandrasekhar limit, above which white dwarfs cannot exist). As residual nuclear fusion shuts down, the star cools and dims at constant radius to become a white dwarf. The luminosity-mass relation is now reversed, as higher mass means higher gravitational compression and smaller radius.
Made of carbon and oxygen surrounded by a thin hydrogen or helium atmosphere, the white dwarf is supported forever by degenerate electron pressure. Because the evolutionary and cooling times of white dwarfs can be calculated theoretically, the oldest of them provides an age measure for the galactic disk, and this measure accords well with that found from open clusters. See also: White dwarf star
High-mass evolution
As the mass of a star increases, so does the mass of its core. The Sun will turn into a white dwarf of around 0.55 solar mass. At roughly 9 solar masses, the core reaches the Chandrasekhar limit, and the star cannot become a white dwarf. At first, the evolution of high-mass stars proceeds similarly to that of stars of lower mass. As high-mass stars use their core hydrogen, they too migrate to the right on the HR diagram, becoming not so much brighter but larger, cooling at their surfaces, and turning into supergiants. Below about 40 solar masses, they become class M red supergiants, losing huge amounts of mass through immense winds. Some (depending on mass) stabilize there by the start of helium fusion; others loop back to become blue supergiants. Above 60 solar masses, so much mass is lost through winds that the stars do not make it much past class B, stalling there as helium fusion begins (Fig. 7). As lower-mass supergiants—as well as bright giants—pass through the middle of the diagram, some become Cepheid variables. See also: Cepheid variables; Supergiant star
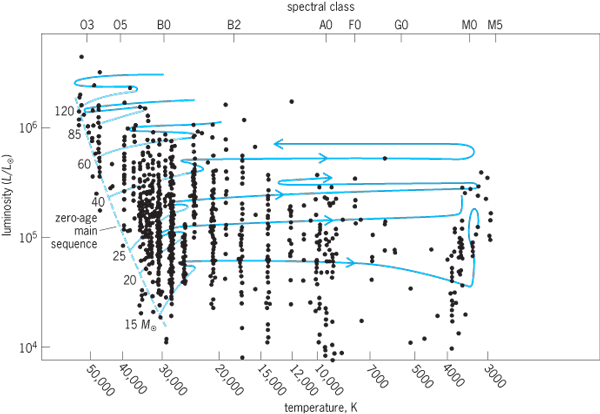
Though almost all these supergiants vary to some extent, the most massive become unstable and undergo huge eruptions. In 1846, Eta Carinae, near 100 solar masses (and a probable double star), brightened to match Sirius and Canopus, lost more than a solar mass, and then faded to near the edge of naked-eye vision (Fig. 8). Such luminous blue variables (LBVs), plus similar lesser lights, are losing their hydrogen envelopes and may be turning into helium-rich Wolf-Rayet stars, highly luminous stars that have been almost completely stripped of their outer hydrogen. Though the sequence is not clear, the luminous blue variables probably first turn into the nitrogen-rich WN variety (whose nitrogen has been enriched through hydrogen fusion by the carbon cycle) and then into the carbon-rich WC species (the carbon from more advanced helium fusion). See also: Wolf-Rayet star
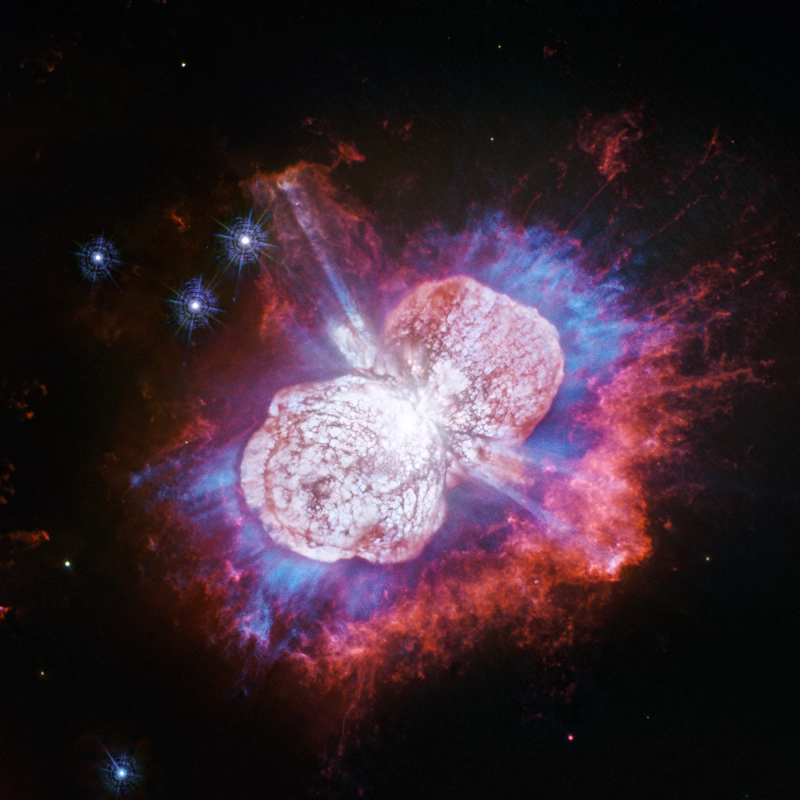
While intermediate-star fusion stops at carbon and oxygen, supergiants continue onward. The carbon-oxygen core shrinks and heats to the point where carbon fusion can begin, and then carbon and oxygen convert to a more complex mix dominated by oxygen, neon, and magnesium. Helium fusion now continues in a surrounding shell that is nested within a shell that is fusing hydrogen. Once carbon burning has run its course, the unsupported oxygen-neon-magnesium core shrinks and heats, and now it is carbon burning's turn to move outward into a shell. When hot enough, the oxygen-neon-magnesium mix fires up to burn to one dominated by silicon and sulfur. Continuing the process, the developed silicon and sulfur core finally becomes hot enough to fuse in a complex way to iron, with the silicon-burning core being wrapped in oxygen-neon-magnesium-, helium-, and hydrogen-burning shells.
Supernova
Each nuclear fusion stage generates less energy, and because each takes on the role of supporting the star, each lasts a shorter period of time. While hydrogen fusion takes millions of years, the iron core is created from silicon fusion in a matter of weeks. Iron cannot fuse and produce energy. The core, about 1.5 solar masses and the size of Earth, suddenly collapses. The iron atoms are broken back to neutrons and protons. Under crushing densities, free electrons merge with protons to make yet more neutrons. When the collapsing neutron core hits nuclear densities of 1014 g/cm3 (1014 times the density of water), it bounces violently, and the rebound tears away all the outer layers.
From the outside, the observer sees the explosion as a type II supernova, which typically can reach an absolute magnitude of −18 (Fig. 9). Temperatures within the exploding layers are high enough that a great variety of nuclear reactions, from equilibrium fusion of one element into another to rapid neutron capture, create all the chemical elements up to and beyond uranium. The optical glow eventually comes mainly from the manufacture of a tenth or so of a solar mass of radioactive nickel, 56Ni, which quickly decays to radioactive cobalt and then to stable iron, 56Fe, which gets blasted into the cosmos along with everything else. See also: Radioactivity
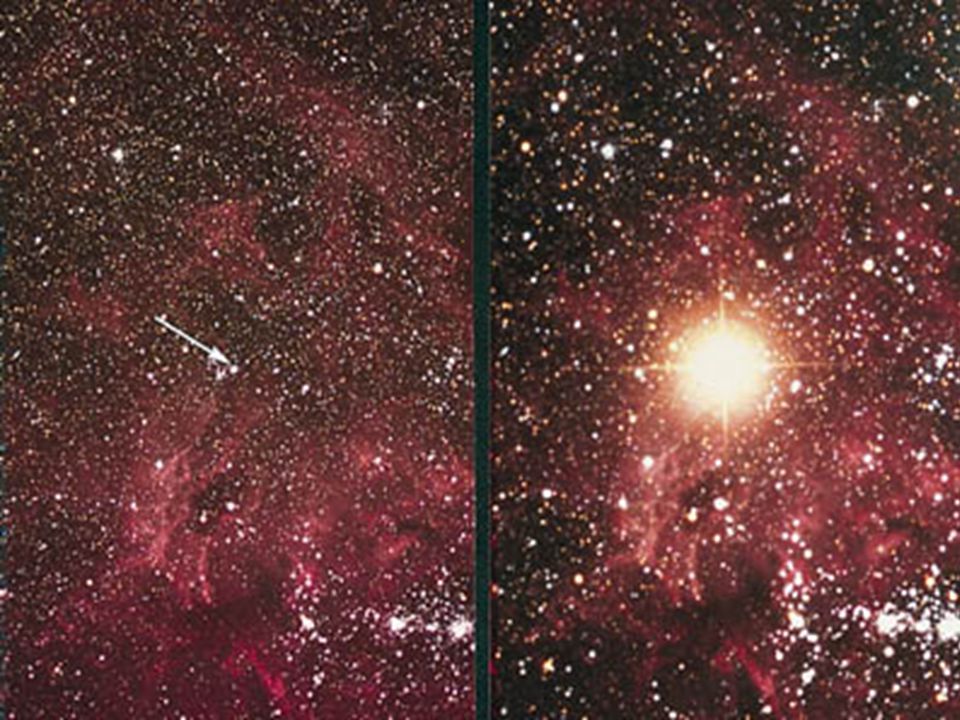
The nature of the rebound is not fully understood. By itself, the rebound does not account for the explosion of a supernova. However, the merger of core protons and electrons generates vast numbers of near-massless neutrinos. Nearly everything is transparent to neutrinos. Those that are created in the solar core by the proton-proton chain go right through the Earth. The densities around the rebounding neutron core, however, are so high that the neutrinos are trapped and likely aid in shoving the surrounding shells and envelope outward. The outflowing neutrinos carry away 100 times more energy than does the blast itself. For a brief moment, the power output of a single supernova equals that of the rest of the stars in the visible universe. At the highest progenitor masses, supernovae are believed to eject their energy in bipolar jets, leading to longer-duration gamma-ray bursts (GRBs). The progeny of O-type stars, supernovae are rare, occurring in the Milky Way Galaxy at a rate of only one or two per century, so the odds of one being close to Earth are fortunately small. See also: Gamma-ray burst; Neutrino; Supernova
Supernova remnants
The debris of the explosion, called the supernova remnant (Fig. 10), is highly enriched in iron and other heavy elements and expands into space for centuries. The supernova shock wave energizes interstellar space, heats vast bubbles of gas to hundreds of thousands of degrees, and helps compress interstellar matter, triggering new star formation. The heavy elements become incorporated into molecular clouds. Most of the Earth's composition came from previous generations of supernovae, each one contributing perhaps a mountain's-worth of mass. Accelerated by the shock, ambient interstellar electrons, protons, and heavier nuclei approach the speed of light and speed through the universe as cosmic rays. See also: Cosmic ray; Crab Nebula; Interstellar matter; Nebula; Shock wave
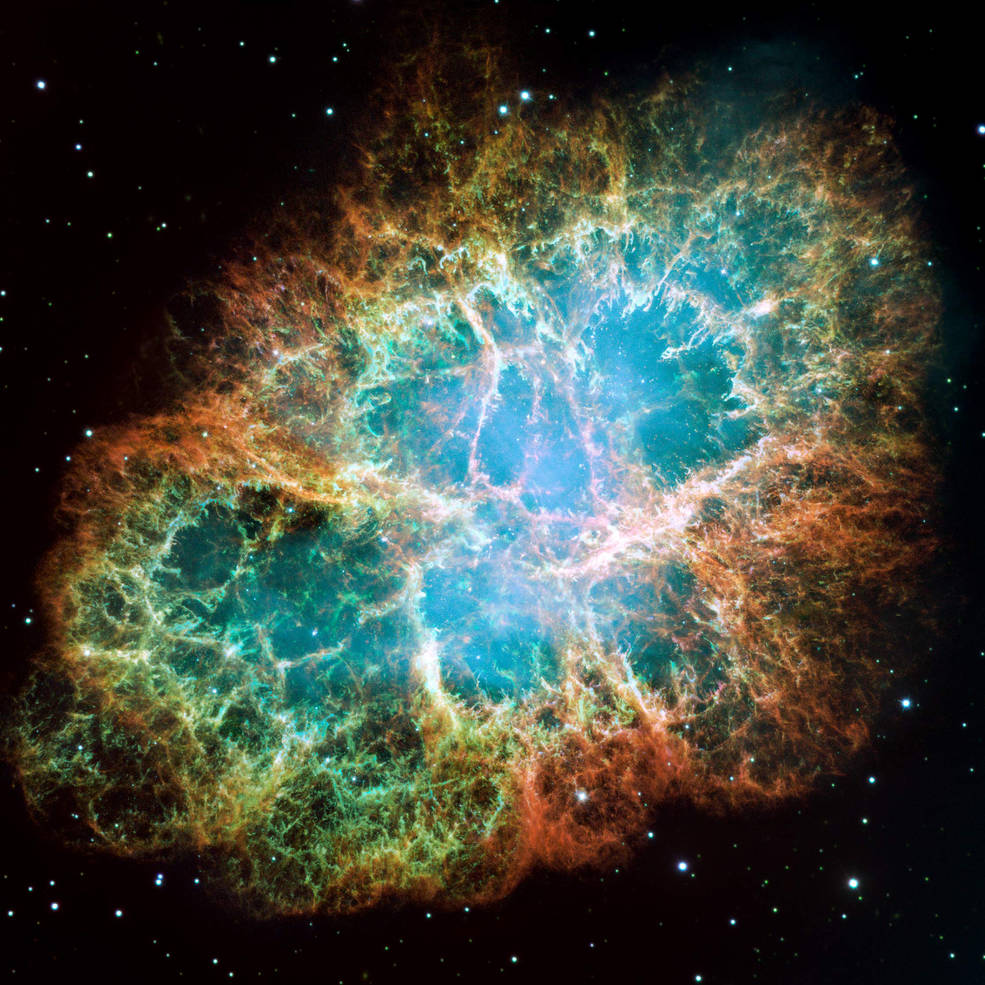
Exposed by the explosion is a compact, hot object known as a neutron star. Only 25 km (15 mi) across, the neutron star is stabilized by the pressure of neutron degeneracy. Conservation of angular momentum makes the little star spin dozens of times per second, with its magnetic field compacted to a trillion or more times stronger than Earth's. Radiation beams out along a tilted, wobbling magnetic axis, and if Earth is in its path, a “pulse” of radiation is observed—the neutron star now being termed a pulsar. As the neutron star radiates away its energy, it spins more slowly and finally disappears from view. The most highly magnetized neutron stars, called magnetars, can have magnetic field strengths greater than 100 trillion times that of Earth, and behind phenomena including anomalous x-ray pulsars (AXPs) and soft gamma-ray repeaters (SGRs), the latter occasionally releasing bursts so powerful that they can affect the Earth's atmosphere. The Milky Way Galaxy should contain more than 100 million quiet, near-invisible neutron stars. Off-center detonation in the supernovae that created neutron stars may give them kicks that can send them off at speeds far higher than those of most of a galaxy's stars. See also: Neutron star; Pulsar
Beyond the Chandrasekhar limit of 1.4 M, electron degeneracy pressure cannot stabilize a white dwarf, and it must collapse. Neutron stars are close to this limit and are themselves similarly limited to around 3 solar masses. Stars at the upper end of the main sequence are expected to create iron cores that exceed even this limiting mass. The dense remains cannot stabilize, and therefore they too must collapse. When such a collapsing star passes a critical radius at which the escape velocity is about that of light, it become invisible, and a black hole is born. See also: Black hole
Double-star evolution
Double (binary) stars are common, with more than half the galaxy's stars having at least one companion. If the components of a binary are well separated by tens or hundreds of astronomical units, the stars evolve separately as if each were single. If the stars are close enough together, however, with the critical limits being dependent on mass, they can profoundly affect each other and their courses of evolution. See also: Astronomical unit
A binary is first made of a pair of dwarf stars. The more massive evolves first into a giant or even a supergiant. If the two are close enough to start with, the growing giant can be affected by the smaller star. As the giant swells, it approaches a teardrop-shaped tidal surface in which the gravitational pulls from the two stars (including the forces resulting from orbital motion) are effectively equal. If the giant or supergiant actually reaches the zero-gravity surface, mass can flow through the point of the teardrop toward the smaller star. If the two still have sufficient separation, the incoming matter will first flow into a disk around the dwarf, from which the dwarf will accrete mass. If the two are close enough, matter will impact the dwarf directly without forming a disk. In either case, the result is that mass will be transferred, resulting both in interesting spectral activity and in the more-massive star eventually becoming the less massive. Among the most dramatic examples of this kind of behavior is the eclipsing binary Algol, in which a class B dwarf accretes mass from a K giant. Mass loss can also wrap the pair in a common envelope. That and angular momentum losses resulting from the close interaction as well as from interacting magnetic fields can draw the two stars closer together and perhaps even make them merge.
The giant eventually produces a planetary nebula and then becomes a white dwarf. Mass loss has left the white dwarf with almost no hydrogen, perhaps only a thin skin on its surface. If the two stars have been drawn close enough together, the white dwarf can tidally stretch the remaining dwarf (usually a G, K, or M star) to the zero-gravity surface, which can then transfer mass, mostly hydrogen, back to the white dwarf (Fig. 11). Instabilities in the accretion disk, as well as ionization and illumination of the flowing stream by a hot spot created by interacting gases, make the pair a flickering cataclysmic variable (CV). When the fresh hydrogen layer is thick, compressed, and hot enough, it burns explosively via the carbon cycle, with the thermonuclear runaway creating a nova that brightens to absolute visual magnitude MV = −10 or so. Several novae are seen each year, with one of first magnitude every few decades. Because a nova is only a surface phenomenon and does not much affect the stars of the binary, the system settles back into its prior CV behavior, and after 100,000 years may produce a nova explosion again. See also: Cataclysmic variable; Nova
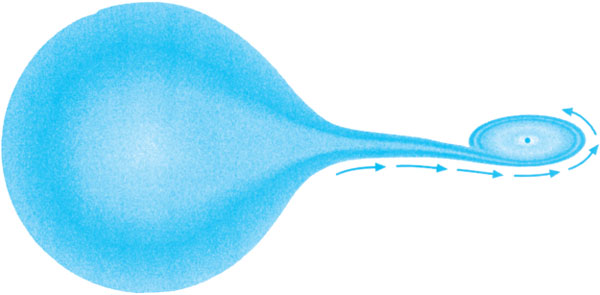
In wider dwarf–white dwarf combinations, the ordinary dwarf will eventually begin its own evolution and become a giant. If the giant received mass from its companion when it was a dwarf (perhaps through a wind), the giant may have been contaminated with by-products of nuclear fusion and appear as a barium star, anomalously rich in barium and other elements. As the giant grows, it passes its mass to the white dwarf through its vigorous wind or via an accretion disk. The hot white dwarf, or a hot boundary layer between an accretion disk and the white dwarf's surface, now gives the binary dual characteristics, those of both a hot and a cool star at the same time. These symbiotic stars can vary dramatically, and the transferred hydrogen can erupt into long-term fusion reactions. See also: Symbiotic star
White dwarf supernovae
There are two quite different kinds of supernovae. Type II supernovae have hydrogen in their spectra. They occur in galactic disks, where young high-mass stars reside, and are produced by iron-core collapse. Type I events, however, do not exhibit spectral hydrogen. They are divided into three categories: Type Ia supernovae display an absorption line of ionized silicon, whereas those of type Ib exhibit helium instead of silicon, and those of type Ic have neither, or weak helium at most. While Ib and Ic supernovae are also confined to the disks of host galaxies, Ia supernovae occur in both disks and galaxy haloes, where there are no high-mass stars. Tycho's Star of 1572 CE and possibly Kepler's Star of 1604 CE were type Ia supernovae.
The Ib variety (and some of the Ic) is probably produced by core collapse in massive hydrogen-poor Wolf-Rayet stars. White dwarfs in double systems are obvious candidates for type Ia. The more massive the receiving white dwarf, the more quickly the infalling fresh hydrogen reaches the nova flash point. If the white dwarf is massive enough, the interval between successive novae becomes less than the time over which the novae have been observed, and the novae become recurrent. For example, RS Ophiuchi and its cohort produce a nova every 20 years or so. Each nova leaves a little residual matter, so the white dwarf increases in mass. If it is pushed over the Chandrasekhar limit, the star must catastrophically collapse and burn, producing a type Ia supernova. A second scenario that might produce type Ia supernovae is the merger of the stars in a double white dwarf system in which the sum of the masses exceeds the Chandrasekhar limit. Type Ia supernovae are even brighter than core-collapse type II events, and can reach MV = −19. Type Ia supernovae are exceeded in brightness only by rare core-collapse events that are probably related to GRBs. Type Ia supernovae also deposit more iron into the cosmos than do the type II, typically three-tenths of a solar mass. All the iron in the universe is made by supernovae. See also: Iron
Though the origins of type Ia explosions are not fully circumscribed, the supernovae have such predictable absolute magnitudes that they can be used to measure distances to distant galaxies and determine not just the Hubble constant but also more subtle expansion characteristics of the universe. See also: Accelerating universe; Cosmology
Heavy-element enrichment
The overall result of stellar evolution is that stars become giants that then turn into white dwarfs, or intrinsically more massive stars become supergiants that then create neutron stars or black holes. Over the course of this evolution, stars feed huge quantities of enriched matter back into the star-generating clouds of interstellar space. Later generations of stars therefore have more heavy elements than do earlier generations. Computer modeling of the chemical composition observed, starting with the hydrogen and helium of the big bang, can closely replicate the chemical composition of the Sun, for instance, providing powerful evidence that the theories are correct. The Earth, made almost entirely of heavy elements, is a distillate of solar gases, and exists only as a result of the combined action of the stars. See also: Big bang theory