Key Concepts
The Sun and the bodies moving in orbit around it. The most massive body in the solar system is the Sun, an average single star that is itself in orbit about the center of the Milky Way Galaxy. Nearly all of the other bodies in the solar system—the terrestrial planets, outer planets, asteroids, and comets—revolve in orbits about the Sun (Fig. 1). Moons revolve around the planets; in addition, the giant planets have orbiting rings. See also: Milky Way Galaxy; Orbital motion; Planet; Sun
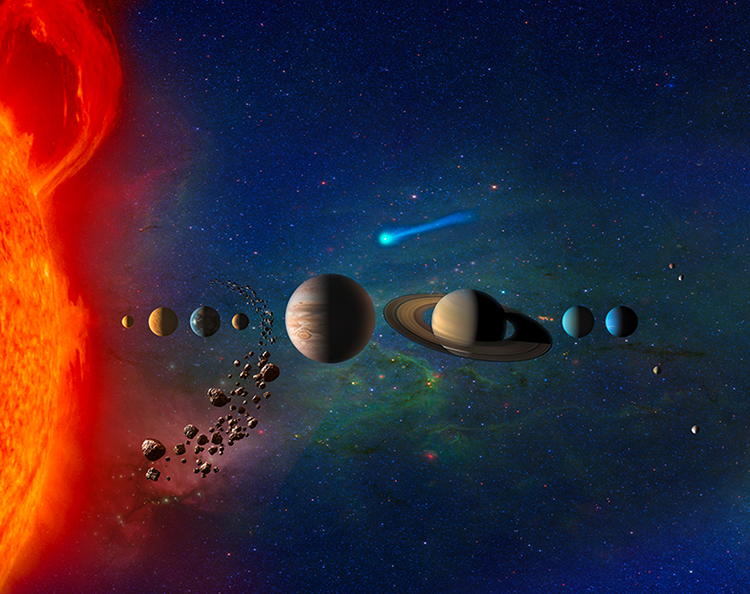
Well-supported models describe how the solar system arose from a rotating cloud of gas and dust starting some 4.567 billion years ago. The cloud collapsed to form a flattened disk (the solar nebula), in which the Sun and other bodies formed. The bulk of the gas in the solar nebula moved inward to form the Sun, while the remaining gas and dust formed most of the other bodies in the solar system by accumulation, proceeding through collisions of intermediate-sized bodies called planetesimals. See also: Collision (physics)
Our solar system is not a unique entity in the universe. Since the 1990s, exoplanetary solar systems (or exosolar systems) have been found in abundance around many other stars in the Milky Way Galaxy. These exoplanetary systems consist of combinations of worlds similar to those found in our solar system, including gas giants (like Jupiter and Saturn) and ice giants (like Uranus Neptune), along with worlds unlike those in our solar system, such as super-Earths, which are massive, rocky planets a bit larger than Earth. Smaller, rocky, terrestrial planets with sizes and compositions similar to Earth are also increasingly being discovered. See also: Exoplanet; Universe
Solar system composition
Sun
The Sun is a gaseous sphere with a diameter of about 1.39 million km (864,000 mi), composed primarily of hydrogen and helium, with small amounts of the other elements. The Sun is just one of about 100 billion stars in the Milky Way Galaxy. The solar system lies in the disk of the Galaxy and moves around the galactic center about once every 200 million years on a circular orbit with a radius close to 30,000 light-years (about 3 × 1017 km or 2 × 1017 mi). The Sun is somewhat peculiar in that it is a single star, unaccompanied by another star; most stars in the disk of the Galaxy are in double or multiple systems, in which two or more stars orbit about their common center of mass. The Sun's mass of 2 × 1030 kg (4.4 × 1030 lb), however, is quite typical; stellar masses range from about 0.08 to potentially hundreds of times the Sun's mass, though the great majority of stars are broadly similar in mass (and hence in other general characteristics) to the Sun. See also: Binary star; Helium; Hertzsprung-Russell diagram; Hydrogen; Light-year; Star; Stellar evolution; Stellar population
Terrestrial planets
The terrestrial planets (Mercury, Venus, Earth, and Mars) are the closest to the Sun, with orbital distances from their host star ranging from 0.39 AU for Mercury to 1.5 AU for Mars. [One astronomical unit (AU), the average distance from Earth to the Sun, equals 150 million km or 93 million mi.] The terrestrial planets are composed primarily of silicate rock (mantles) and iron (cores). The Earth is the largest terrestrial planet, with an equatorial diameter of 12,742 km (7,918 mi) and a mass of 5.972 × 1024 kg (1.317 × 1025 lb); Mercury is the smallest, with a mass 0.055 times that of Earth. See also: Astronomical unit; Earth; Earth's core; Iron; Mars; Mercury (planet); Silicate minerals; Venus
Outer planets
The outer planet region begins with Jupiter at a distance of 5.2 AU from the Sun. The outer planets are subdivided into the gas giant or Jovian planets (Jupiter and Saturn) and the ice giant planets (Uranus and Neptune). By far the largest planet is Jupiter, with a mass 318 times that of the Earth, followed by Saturn (95 Earth masses), Neptune (17 Earth masses), and Uranus (15 Earth masses). Jupiter and Saturn are composed primarily of hydrogen and helium gas, like the Sun, but with rock and ices, such as frozen water, methane, and ammonia, concentrated in their cores. Uranus and Neptune also have rock and ice cores surrounded by envelopes with smaller amounts of hydrogen and helium. See also: Jupiter; Neptune; Saturn; Uranus
Dwarf planets
Pluto, discovered in 1930 by U.S. astronomer Clyde Tombaugh at the Lowell Observatory in Arizona, was long considered to be the outermost, ninth planet. As observations of Pluto improved over the intervening decades, however, the size of Pluto became smaller and smaller; we now know that Pluto is a third smaller in diameter than the Earth's Moon. In 2006, the International Astronomical Union (IAU), an organization charged with astronomical naming conventions, demoted Pluto from being a planet. However, this demotion was not based solely on Pluto's small size, but largely on the discovery since the 1990s of numerous other similar-sized bodies in the outer solar system. One body in particular, called Eris, was discovered by U.S. astronomer Michael Brown in 2005. Eris is ever so slightly smaller than Pluto, but is more massive, and orbits the Sun at a mean distance of 65 AU, compared to 39 AU for Pluto. The IAU, faced with declaring all of these similar-sized bodies to be “planets,” voted in August 2006 to create a new class of objects called dwarf planets. The five recognized dwarf planets to date are Pluto, Eris, Haumea, and Makemake—all located in orbits beyond Neptune, though Pluto's orbit does occasionally take it closer to the Sun than Neptune—as well as Ceres, located in the asteroid belt, described next. See also: Ceres; Pluto
Asteroids
Asteroids are rocky objects too small to be considered planets and usually have lumpy, irregular shapes. Many known asteroids are about 1 km (0.6 mi) in radius, although some have radii of hundreds of kilometers. Tens of thousands of asteroids populate the region between Mars and Jupiter, known as the asteroid belt. Other groups of asteroids, known as Trojan asteroids, precede and follow the planet Jupiter in its orbit at so-called Lagrangian points where the gravitation from the gas giant is balanced by the Sun's attraction. Neptune also has a smaller collection of Trojans, and Uranus has at least two such asteroids, though none have yet been confirmed in association with Saturn's orbit. See also: Asteroid; Trojan asteroids
Comets
Comets are icy bodies (akin to dirty snowballs) with diameters of the order of 10 km (6 mi). In contrast to the orbits of most planets, the orbits of comets often are highly elliptical and have large inclinations that take them far above and below the plane near which the planets orbit, called the ecliptic. The region well beyond Pluto's orbit is populated with a very large number of comets, perhaps a trillion, out to a limiting distance of about 100,000 AU, at which point external forces (created by nearby stars, passing molecular clouds, and the Milky Way Galaxy itself) disturb any body that is trying to stay in a stable orbit around the Sun. See also: Comet; Ecliptic
Oort Cloud and Kuiper Belt
The huge volume of space beyond Pluto that is still considered part of the solar system is called the Oort Cloud. The distribution of comets in the Oort Cloud is uncertain; there may be an inner cloud extending outward to about 20,000 AU and an outer cloud beyond that. In addition, comets have been detected orbiting in the plane of the solar system at distances of about 50 AU and beyond; this flattened distribution is called the Kuiper Belt (KB), named for the Dutch-born U.S. astronomer Gerard Kuiper who vaguely suggested its existence in 1951. The largest KB objects can now be observed from Earth, with diameters in the range from 100 to more than 2300 km (60 to 1480 mi); these largest KB objects are also often called trans-Neptunian objects (TNOs). Pluto and Eris are the two largest bodies in this family of icy objects, the very largest of which are now called dwarf planets, as previously discussed (Fig. 2). See also: Kuiper Belt
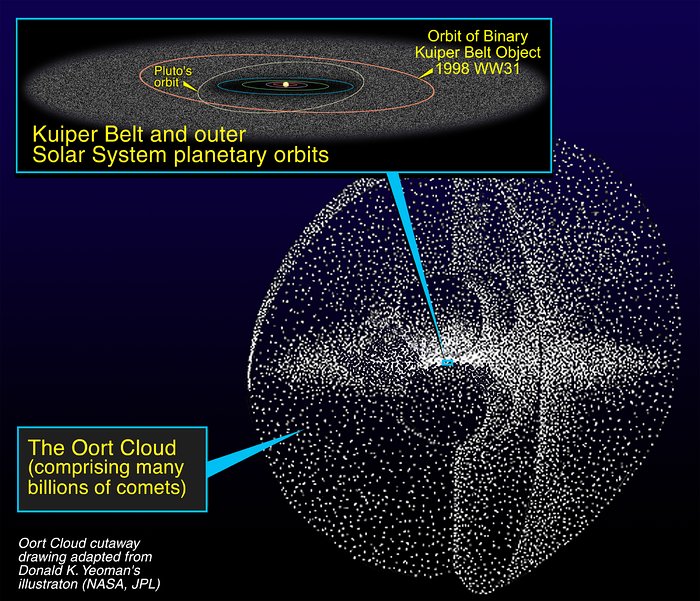
Most of the KB bodies, however, are much smaller than the dwarf planets, and they make up several distinct populations, generally orbiting well beyond Neptune's 30-AU distance. The classical KB consists of comets with mean distances from the sun of about 45 AU, with nearly circular orbits that lie close to the ecliptic. The scattered KB disk consists of comets that orbit even farther from the Sun, in orbits that are elliptical and highly inclined to the ecliptic. Other KB objects orbit in so-called resonant orbits, whose orbital periods are synchronized with that of Neptune; for example, Pluto orbits the Sun twice during every three orbits of Neptune. Comets that orbit closer in will cross the orbit of Neptune, and are thus in danger of being gravitationally kicked inward or outward. Comets that enter the inner solar system are eventually evaporated by solar radiation, leaving behind interplanetary dust particles and perhaps rocky cores similar to certain asteroids. See also: Comet Hale-Bopp; Orbital motion
Moons
Natural satellites (also called moons) orbit around all of the planets except Mercury and Venus. The Earth and its rocky Moon are unusual in that the Moon is about one-fourth the size of the Earth; nearly all other satellites are much smaller than their planet, with the exception of Pluto's satellite Charon, which is half the size of Pluto. While Mars has only two tiny, rocky satellites, the Voyager, Galileo, Cassini, New Horizons, and Juno missions, as well as ground-based observations, have shown that the giant planets all have extensive systems of satellites (Jupiter has at least 79). Each giant planet also has a number of rings of particles whose orbits are interspersed with and controlled by the planet's innermost satellites. See also: Moon; Satellite (astronomy)
Origin of the solar system
A number of imaginative theories of the origin of the solar system have been presented in the last several hundred years, but nearly everyone who is presently working on solar system cosmogony agrees on one fundamental concept, advanced in 1796 by French astronomer and mathematician Pierre Simon de Laplace. This concept, the nebular hypothesis, holds that the Sun and the rest of the bodies in the solar system formed from the same rotating, flattened cloud of gas and dust, which as previously mentioned is called the solar nebula. The nebular hypothesis at once explains the gross orbital properties of the solar system: all planets orbit (and most rotate) in the same sense as the Sun rotates, with their nearly circular orbits being confined largely to the ecliptic, the single plane almost perpendicular to the Sun's rotation axis. See also: Stellar rotation
While the grand experiment that led to the formation of the solar system can never be repeated, present-day regions of star formation in the Milky Way Galaxy can be observed to gain insight into the processes involved in the formation of stars similar in mass to the Sun. Assuming that present-day star formation proceeds much as it did 4.567 billion years ago, this approach should yield many constraints on the general process. These observations confirm the stellar implications of Laplace's nebular hypothesis: very young stars (protostars) are indeed found to be embedded in dense clouds of gas and dust that often show evidence of flattening and rotation. While understanding of the formation of the solar system is almost certain to undergo further evolution, the following provides a detailed framework in which cosmogonists are working. See also: Molecular cloud; Protostar
Collapse phase
The solar nebula was produced by the collapse of a dense interstellar cloud (Fig. 3a), composed primarily of molecular hydrogen (about 77% by mass) and helium gas (about 21%), with about 2% of the cloud mass being in the form of the other elements, mostly frozen into dust grains. Elements heavier than hydrogen and helium were formed by nucleosynthesis in previous generations of stars and then ejected into interstellar clouds through events such as supernovae. See also: Nucleosynthesis; Supernova
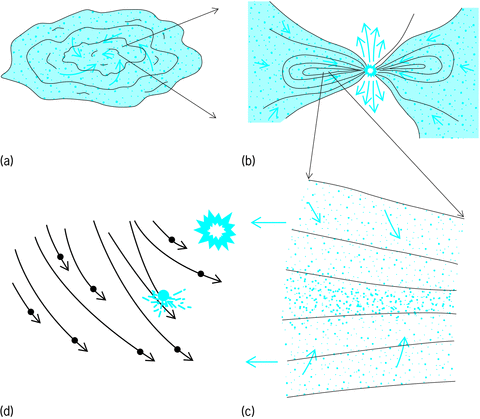
Radio telescopes have shown that dense interstellar clouds exist with masses comparable to that of the Sun, containing about 10,000 to a million molecules/cm3, and with quite low temperatures, about 10 K (−263°C or −441°F). Because of their low temperatures, gas pressure generally is not sufficient to support these clouds against their own self-gravity, which tries to pull the cloud into a smaller configuration. Many clouds appear to be supported primarily by magnetic fields; however, magnetic field support requires a continual contraction of the largely neutral cloud past the field lines, and eventually magnetic forces can no longer support the increasingly dense cloud. At this point, dense clouds enter the collapse phase, where supersonic inward motions develop that lead to the formation of a stellar-sized core at the center of the cloud in about 100,000 to a million years. Some of the gas in the cloud continues to fall onto this protostellar core, where it is suddenly stopped and its kinetic energy is converted into thermal energy. This heat leaves the cloud as infrared radiation; infrared telescopes have shown that many dense cloud cores contain deeply embedded young stars. See also: Energy; Gravitational collapse; Infrared astronomy; Interstellar matter; Radio astronomy; Stellar evolution
Solar nebula
Because of the conservation of angular momentum, not all of the in-falling gas and dust in a rotating cloud falls directly onto the central protostar. Instead, a disklike solar nebula forms, where the inward pull of gravity is expended in maintaining the rotational motion of the nebula. If too much angular momentum is present in the initial cloud, the collapse process is likely to result in the formation of a double or multiple star system instead of a single star and a planetary system; if extremely little angular momentum is present, only a single star forms. See also: Angular momentum; Gravity
Because the Sun contains 99.9% of the mass of the solar system but only about 2% of the angular momentum, the planetary system has considerably more angular momentum per unit mass than the Sun. This discrepancy is more than can be accounted for by forming the Sun out of the lowest-angular-momentum portions of a dense cloud, or by allowing for the fact that during the life of the solar system, the Sun has lost substantial angular momentum through magnetic braking associated with the solar wind, the radiation constantly streaming off the Sun. See also: Solar wind
The solution to this angular momentum problem appears to lie in substantial evolution of the solar nebula after its formation. In order to have enough angular momentum to lead to the formation of a planetary system, much of the mass of the newly formed solar nebula must reside in the disk rather than in the early Sun. The disk must then evolve in such a way as to transfer mass inward to feed the growing Sun, while transporting outward the excess angular momentum that is undesired by the Sun but required for the planets. While this sort of evolution may appear to be contrived, if not miraculous, it is actually to be expected on very general grounds for any viscous disk that is undergoing a loss of energy, as the solar nebula will, through radiation to space. Several different physical mechanisms have been identified that potentially are capable of driving solar nebula evolution: turbulence caused by convective instability or velocity shear, gravitational torques between spiral arms and clumps in the nebula, and magnetic torques associated with a nebular magnetic field generated by a magnetorotational instability. These mechanisms are likely to lead to evolution of the bulk of the nebula gas inward onto the protosun (Fig. 3b), on a time scale on the order of 100,000 to 10 million years. Magnetic fields and gravitational torques are the currently favored drivers of nebula evolution.
Planetesimal formation
The portion of the nebula that is to form the planets must decouple from the gaseous nebula to avoid being swallowed by the Sun. This decoupling occurs by the process of coagulation of dust grains through mutual collisions; when solid bodies become large enough (roughly kilometer-sized), they will no longer be tied to the nebula through Brownian motion (as is the case with dust grains) or gas drag (as happens with smaller bodies). See also: Brownian movement
The interstellar dust grains that eventually form the solid bodies in the solar system start out having sizes on the order of 0.1 micrometer. These minuscule particles must stick together in order to begin the planet formation process; sticking can be caused by van der Waals forces. Coagulation is enhanced by the increase in the spatial density of dust grains, which occurs as the dust grains sediment down through the gaseous nebula to form a dense subdisk composed largely of solid particles (Fig. 3c). Both dust grain sedimentation and coagulation are inhibited by the vigorous stirring that is to be expected in a highly turbulent nebula (the latter because grains may hit each other at relative velocities that are high enough to produce fragmentation rather than coagulation), but once the turbulence dies down, sedimentation and coagulation can produce centimeter- to meter-sized bodies in about 1,000 to 10,000 years. See also: Intermolecular forces; Van der Waals equation
The next phase of growth has long been thought to involve a collective gravitational instability of the dust subdisk that would rapidly produce bodies of kilometer size or larger, termed planetesimals. However, this instability may be prevented by turbulence induced by the difference in orbital speed between the gaseous nebula and the dust subdisk. (The gas orbits the Sun somewhat more slowly than the solid bodies because the gaseous disk is partially supported against the Sun's gravity by gas pressure.) If the gravitational instability is prevented, then growth to planetesimal size may occur through further collisional coagulation; gas drag preferentially slows down smaller bodies, producing relative motions between larger and smaller bodies that can lead to collisions. In this case, growth to kilometer-sized planetesimals is expected to occur in about 10,000 years. There continues to be much debate as to whether or not dust disk instability was actually involved in growth to this size.
Planetary accumulation
About a trillion kilometer-sized planetesimals are needed to form just the terrestrial planets; significantly greater numbers of similarly sized bodies would be needed to form the giant planets. These planetesimals are already roughly the size of many asteroids and comets, suggesting that many of the bodies are simply leftovers from intermediate phases of the planet formation process. This process unfolds in what is known as a protoplanetary disk surrounding the young host star (Fig. 4).
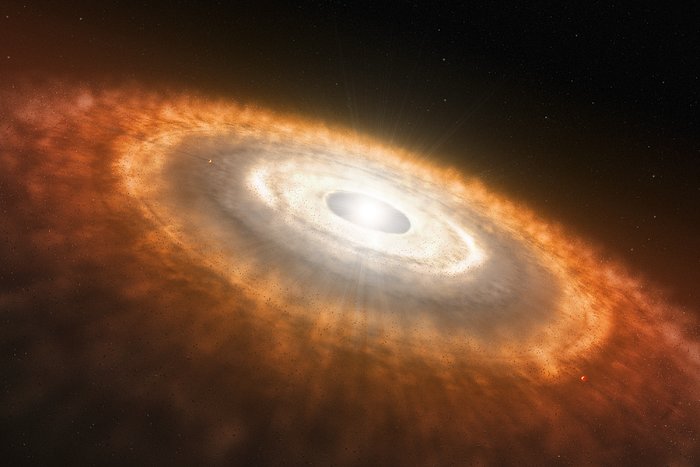
Planetesimals are massive enough for gravitational forces to determine their collision probabilities; self-gravity is also needed to prevent debris from escaping following collisions. The subsequent growth of the planetesimals through gravitational accumulation (Fig. 3d) occurred in two distinct phases. In the first phase, planetesimals grew by accumulation of other planetesimals at essentially the same distance from the Sun. Once the nearby planetesimals were all swept up, this phase ended. The first phase lasted about 10,000 years in the inner solar system and produced planetesimals about 500 km (300 mi) in size. This phase is likely to have been characterized by the runaway growth of relatively few bodies, because once one body becomes larger than its neighbors (as must happen after any collision occurs), its increased gravitational pull increases the chances that another body will collide with it, and so on.
In the second phase, accumulation requires bodies at significantly different distances from the Sun to collide. This can happen only if the planetesimal orbits become highly elliptical and hence intersecting. Gravitational forces between the orbiting planetesimals can produce elliptical orbits, but only over relatively long time periods; about 10 million to 100 million years are required for accumulation to proceed by this means in the inner solar system. The second phase is currently thought to have proceeded differently from the first phase, with collisions occurring primarily between more or less equal-sized bodies, rather than the highly unequal-sized collisions that occur in a runaway accumulation process. The second phase may then have involved violent collisions between planetary-sized bodies, a spectacular finale to the entire planet formation process. A glancing collision between a Mars-sized and an Earth-sized body appears to be the best explanation for the formation of the Earth-Moon system; debris from the giant impact would end up in orbit around the Earth and later form the Moon.
Astronomical observations of young stars similar to the Sun imply that the gaseous portion of the solar nebula was removed some time between 100,000 and 10 million years after the Sun began to form. While most of the gas presumably was added to the Sun through nebula evolution, the residual nebula gas and dust were probably removed from the solar system by the early solar wind, which had a mass loss rate roughly a million times larger than at present (Fig. 3b). The final formation of the terrestrial planets, lasting 10 million to 100 million years, then occurred in the absence of appreciable nebula gas, which explains the absence of significant amounts of hydrogen and helium in the inner solar system. However, formation of the giant planets must have occurred prior to the loss of the nebula gas; otherwise their rock and ice cores would not have been able to capture the gas needed to account for their present compositions. Formation of the giant planets within about a million years appears to require that the second phase of planetesimal accumulation proceeded through runaway accretion all the way to bodies about 10 Earth masses in size in the outer solar system. The satellite systems of the giant planets were largely formed in minisolar nebulae orbiting around each protoplanet, through processes that were similar to those described for planet formation.
Forming gas giant planets by the two-step process requires about 10 million years for a 10-Earth-mass core to form and then accrete a massive gaseous envelope, which may be longer than the life of typical gaseous disks. The alternative means for forming the gas giant planets is much more rapid, requiring only about 1,000 years for a gravitational instability of the gaseous nebula to produce a massive clump of gas and dust. The dust will settle to form a core at the center of the clump on a similar time scale. If gas giant planets form by the latter mechanism, then even the youngest stars will show evidence of Jupiter-mass companions; while if the two-step mechanism predominates, most young stars will not be old enough to have such companions. The formation of ice giant planets could occur through the disk instability mechanism if the solar nebula was born in a region of high-mass stars. Their radiation would photoevaporate the disk gas beyond Saturn's orbit as well as the gaseous envelopes of the protoplanets orbiting there.
Exoplanetary systems
Extensive support for the implications of the nebular hypothesis for planetary formation has come from the detection of planetary systems in various stages of formation around other stars. Furthermore, if the basic theory of solar system formation is correct, planetary systems should not be rare in the Milky Way Galaxy. Indeed, all evidence to date indicates that planets outnumber stars in the Milky Way by a substantial margin, suggesting a galaxy teeming with trillions of exoplanets (Fig. 5). See also: Exoplanet
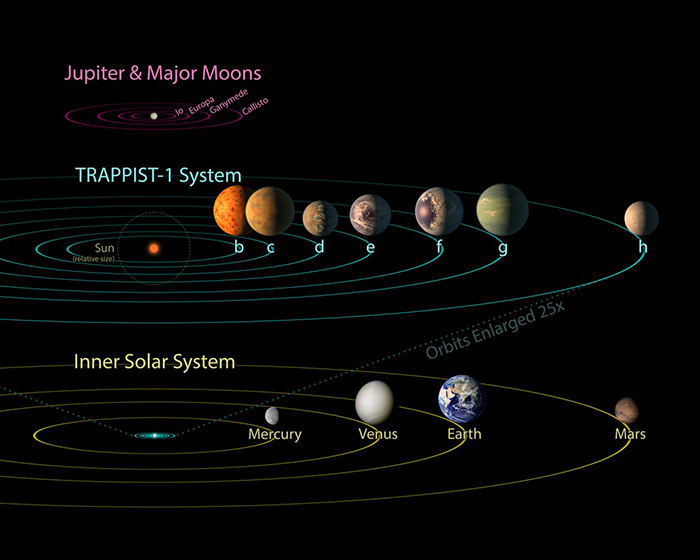
The new era in the study of planetary origins began in 1995, when Swiss astrophysicists Michel Mayor and Didier Queloz found evidence for a Jupiter-mass planet in orbit around the Sun-like star 51 Pegasi. Since then, several thousand other exoplanets have been discovered, with orbital distances ranging from a surprising thousandth of an AU to a thousand AUs. These discoveries appear to show that some planets can migrate inward toward their stars following their formation, pointing to dynamic evolutions of solar systems over time.
Beyond planetary science itself, a prime motivator for deeper studies of the worlds in our solar system as well as exoplanets is the search for extraterrestrial life. Earth remains the only body known in the cosmos that is an abode for biology. The discipline of astrobiology seeks to understand the factors that are required for life to arise, given conditions not necessarily like those found on Earth. The results from the Kepler space telescope, whose primary mission ran from 2009 to 2013, revealed that the frequency of potentially habitable Earth-like planets in our Galaxy should be quite high. Subsequent missions are now locating the most promising exoplanets and exoplanetary solar systems for detailed investigation that might yield critical clues about the origin of life. See also: Astrobiology; Kepler mission