Key Concepts
High-molecular-weight materials composed of repeating subunits; also known as macromolecules, high polymers, giant molecules, and plastics. These materials may be organic, inorganic, or organometallic, and synthetic or natural in origin. Polymers are essential materials for many applications, such as adhesives, building materials, fibers, fabrics, paper, packaging materials, and coatings (Fig. 1). Plastics is the generic and engineering term often used to describe hard and flexible polymers that can be shaped or molded into various objects. Plastics may contain additives for modifying their physical properties and processability. A common example of a plastic is the polyethylene terephthalate (polyester) bottle for packaging water and other beverages. See also: Inorganic and organometallic polymers; Plastics processing; Polyester resins
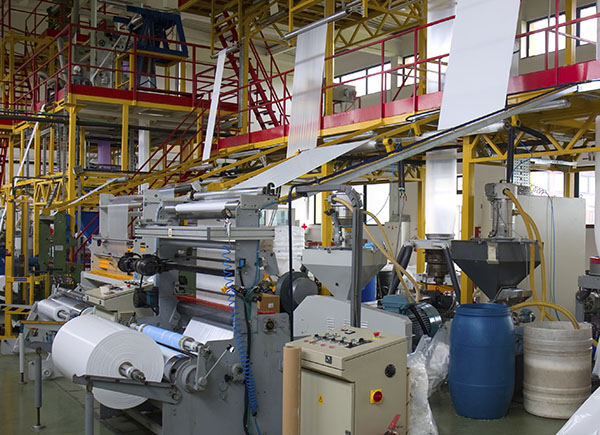
Natural inorganic polymers include diamonds, graphite, sand, asbestos, agates, chert, feldspars, mica, quartz, and talc. Natural organic polymers include polysaccharides (or polycarbohydrates) such as starch and cellulose, nucleic acids, and proteins. Synthetic inorganic polymers include boron nitride, concrete, many high-temperature superconductors, and a number of glasses. Siloxanes or polysiloxanes represent synthetic organometallic polymers. See also: Nucleic acid; Polysaccharide; Protein; Silicone resins
Synthetic polymers offer a savings in energy requirements over other materials such as metals. Their lightness reduces the consumption of fuel in vehicles and aircraft, and they outperform most metals when measured on a strength-per-weight basis. As properties have improved, polymers have been developed which can be readily and economically fabricated, and which can be used for engineering purposes such as gears, bearings, and structural members.
Nomenclature
Since much of polymer science was developed in industry and within a wide range of disciplines, many polymers have both a common name and a structure-based name specified by the International Union of Pure and Applied Chemistry (IUPAC). Many polymers are commonly known by their acronyms. See also: Organic nomenclature
For example, polystyrene or PS is named poly(1-phenylethylene) by the IUPAC, while poly(methyl methacrylate) or PMMA is named poly[(1-metho-xycarbonyl)-1-methylethylene]. See also: Polyacrylate resin; Polystyrene resin
Many companies use trade names to identify the specific polymeric products they manufacture. For example, Fortrel® polyester is a poly(ethylene terephthalate) or PET fiber. Many polymers are generically named, such as rayon, polyester, and nylon.
Composition
Generally, polymers have structures that can be represented by similar or identical repeat units. The repeat units are derived from smaller molecules, called monomers, which react to form the polymer. For example, propylene monomer (structure
1
) and the repeat unit (
2
) it forms in polypropylene are shown below.
With the exception of its end groups, polypropylene is composed entirely of this repeat unit. The number of units (n) in a polymer chain is called the degree of polymerization or DP. See also: Polyvinyl resins
Other polymers, such as proteins, can be described in terms of the approximate repeat unit (
3
)
where the nature of R (a substituted atom or group of atoms) varies. See also: Protein
Primary structure
The sequence of repeat units within a polymer is called its primary structure. Unsymmetrical reactants, such as substituted vinyl monomers (
4
),
react almost exclusively to give a “head-to-tail” product (
5
), in which the R substituents occur on alternate carbon atoms. A variety of head-to-head structures (
6
)
are also possible. Each R-substituted carbon atom is a chiral center (one atom in a molecule attached to four different groups) with different geometries possible. Arrangements where the substituents on the chiral carbon are random are referred to as atactic structures. Arrangements where the geometry about the chiral carbon alternates are said to be syndiotactic. Structures where the geometry about the chiral atom have the same geometry are said to be isotactic. Both syndiotactic and isotactic polymers are stereoregular. See also: Polymer stereochemistry and properties
Stereoregular polymers are produced using special stereoregulating catalyst systems. A series of soluble catalysts have been developed that yield products with high stereoregularity and low chain size disparity. As expected, polymers with regular structures—that is, isotactic and syndiotactic structures—tend to be more crystalline and stronger.
Polymers can be linear or branched with varying amounts and lengths of branching (Fig. 2). Most polymers contain some branching.
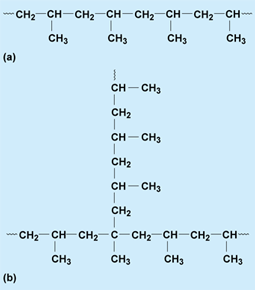
Copolymers are derived from two different monomers, which may be represented as A and B. There exists a large variety of possible structures and, with each structure, specific properties. These varieties include alternating, random, block, and graft (Fig. 3). See also: Branched polymer; Copolymer
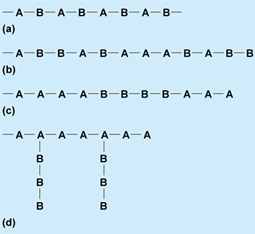
Secondary structure
Secondary structure refers to the localized shape of the polymer, which is often the consequence of hydrogen bonding and other hydrophilic and hydrophobic interactions. (Nonpolar regions tend to clump with other nonpolar regions, while polar regions tend to clump with other polar regions.) Most flexible to semiflexible linear polymer chains tend toward two structures—helical and pleated sheet/skirtlike. The pleated skirt arrangement is most prevalent for polar materials where hydrogen bonding can occur. In nature, protein tissue is often of a pleated skirt arrangement. For both polar and nonpolar polymer chains, there is a tendency toward helical formation with the inner core having “like” secondary bonding forces. See also: Hydrogen bond
Tertiary structure
Tertiary structure refers to the overall shape of a polymer, such as in polypeptide folding. Globular proteins approximate rough spheres because of a complex combination of environmental and molecular constraints, and bonding opportunities. Many natural and synthetic polymers have “superstructures,” such as the globular proteins and aggregates of polymer chains, forming bundles and groupings.
Quaternary structure
Quaternary structure refers to the arrangement in space of two or more polymer subunits, often a grouping together of tertiary structures. For example, hemoglobin (quaternary structure) is essentially the combination of four myoglobin (tertiary structure) units. Many crystalline synthetic polymers form spherulites. See also: Hemoglobin
Synthesis
For polymerization to occur, monomers must have at least two reaction points or functional groups. There are two main reaction routes to synthetic polymer formation—addition and condensation. In chain-type kinetics, initiation starts a series of monomer additions that result in the reaction mixture consisting mostly of unreacted monomer and polymer. Vinyl polymers, derived from vinyl monomers and containing only carbon in their backbone, are formed in this way. Examples of vinyl polymers include polystyrene, polyethylene, polybutadiene, polypropylene (
7
),
and poly(vinyl chloride). See also: Polymerization; Polyolefin resins
The second main route is a step-wise polymerization. Polymerization occurs in a step-wise fashion so that the average chain size within the reaction mixture may have an overall degree of polymerization of 2, then 5, then 10, and so on, until the entire mixture contains largely polymer with little or no monomer left. Polymers typically produced using the step-wise process are called condensation polymers, and include polyamides (
8
), polycarbonates (
9
), polyesters, and polyurethanes (
10
). See also: Polyamide resins; Polyurethane resins
Condensation polymer chains are characterized as having a noncarbon atom in their backbone. For polyamides the noncarbon is nitrogen (N), while for polycarbonates it is oxygen (O). Condensation polymers are synthesized using melt (the reactants are heated causing them to melt), solution (the reactants are dissolved), and interfacial (the reactants are dissolved in immiscible solvents) techniques. The table lists some addition and condensation polymers. See also: Phenolic resin; Polyacrylonitrile resins; Urea-formaldehyde resins
Polymer |
Type of synthesis |
---|---|
Polyesters |
Condensation |
Ureas |
Condensation |
Phenolics |
Condensation |
Polyethylene |
Addition |
Polypropylene |
Addition |
Polystyrene |
Addition |
Poly(vinyl chloride) |
Addition |
Polyacrylonitrile |
Addition |
Polyamides, nylons |
Condensation |
Olefins |
Addition |
Polybutadiene |
Addition |
Styrene-butadiene |
Addition |
Properties
The properties of polymeric materials are determined by their molecular properties and morphology. Polymer morphology in turn depends on the polymerization conditions.
Molecular properties
These include molecular size and weight, molecular structure or architecture, molecular weight distribution, polarity, and flexibility of the polymeric chains (or chain segments between crosslinks in cured or vulcanized polymers). Molecular properties taken together determine the attractive forces between the molecules, the morphology or arrangement of masses of molecules, and the general behavior of the polymer.
Molecular weight
The molecular weight of a particular polymer chain is the product of the number of units times the molecular weight of the repeating unit. Polymerization reactions, both synthetic and natural, generally produce products with varying chain lengths. [The exception is the biochemical reactions that produce enzymes (proteins) and nucleic acids (such as DNA), which have precise molecular weights and chain orientations critical to their function.]
A polymer's varying chain lengths result in a product with a distribution of molecular weights. This molecular weight distribution may be narrow or broad, monomodal or polymodal, depending on the polymerization conditions.
Two statistical averages describe polymers, the number-average molecular weight n and the weight-average molecular weight w. The n is particularly dependent on the number of polymer chains with a specific chain length, while the w is dependent on the size distribution of the polymer chains. The n is determined using procedures that are related to the number of chains of a particular length. Colligative properties are dependent on the number of particles present, and can be used to determine n. These techniques include membrane osmometry, vapor-phase osmometry, ebulliometry (boiling-point elevation), cryoscopy (melting-point depression), end-group analysis, and osmodialysis. Light-scattering photometry is the most often used technique for measuring w.
Since polymers generally greatly increase the viscosity (resistance to flow) of solutions containing them, viscometry is often used to determine the relative molecular weight of polymers. Today, combinations of gel permeation chromatography and light-scattering photometry can be used to determine both average molecular weight and the molecular weight of narrow molecular weight groupings. See also: Light-scattering techniques; Molecular weight; Scattering of electromagnetic radiation; Viscosity
Size
Size is the most important property of polymers. Size allows storage of information (nucleic acids and proteins) and allows polymeric materials to “remember” when reversible movement occurs; in cases where movement is not reversible, polymer structures are a consequence of past treatment such as bending, stretching, melting—any action that distorts or moves polymer chains or segments). For large chains, entanglement occurs, introducing physical crosslinking and enhancing the ability of the polymers to remember and return to original molecular structures after applied stress/strain. An aspect of this remembering involves the need for segments of a polymer chain to accompany adjacent polymer segments. Thus, for space travel applications, even when the vacuum is enough to allow segmental “evaporation” of external polymer chains, some of the chain remains connected, trapped within the interior of the polymer matrix, preventing total removal. Polymeric materials are used extensively on the space shuttles.
Attractive forces
Size also accounts for an accumulation of the interchain (between molecules) and intrachain (within the same molecule) secondary attractive forces. These secondary forces, called van der Waals forces, are composed of London or dispersion forces, dipole-dipole forces, and hydrogen bonding. For a nonpolar polymer, such as polyethylene (
11
),
the attractive forces for each unit are about 2 kcal/mole per repeating unit. For a polyethylene chain 1000 units long, the cumulative dispersion attractive forces would be about 2000 kcal/mole, which is well above the carbon-carbon bond strengths so that even low-molecular-weight polymer chains are nonvolatile. As the temperature is increased, polymers will decompose prior to volatilization. See also: Chemical bonding; Intermolecular forces
For polar polymers, such as poly(vinyl chloride) (
12
),
attractive forces include both dispersion and dipole-dipole forces so that the total attractive forces are proportionally larger than those for polyethylene. Examples of polar polymers include poly(vinyl acetate) (
13
), poly(ethylene glycol) (
14
), and poly(methyl methacrylate) (
15
). For polymers that can take advantage of hydrogen bonding, the attractive forces are even greater. Such polymers include poly(vinyl alcohol), proteins, polysaccharides, nucleic acids, and nylons (
16
). ((( See also: Poly(ethylene glycol)
Hydrogen bonding is so strong in cellulose that cellulose is not soluble in water until the inter- and intrachain hydrogen bonds are broken. Thus, “wood shaping” is often accomplished by breaking the hydrogen bonds by addition of sodium hydroxide. After the wood is shaped, the sodium hydroxide is washed away and the hydrogen bonds form again, allowing the wood to retain a new shape. This principle is similar to that of permanent press clothing, where crosslinks are introduced to retain fabric shape. See also: Cellulose
Morphology
As bonding forces and symmetry increase, the tendency to form regular structures increases, and since the inter-and intrachain forces are inversely proportional to the square of the distance, the chains are able to reside closer together. Polymers often have a combination of ordered regions, called crystalline regions, and disordered or amorphous regions. The amorphous regions contribute to polymers' flexibility and diffusivity of gases and other materials. At low temperatures these regions are rigid, but as the temperature increases there is a temperature range where local or segmental chain mobility occurs. This temperature range is called the glass-phase transition temperature, Tg. Polymers are flexible only above their glass-phase transition temperature.
Regions where polymer chains are aligned in an orderly manner are called crystalline regions. Crystalline regions contribute to strength and resistance to external forces, including chemical attack. Crystalline regions can be melted. At the melting point or range, whole-chain movement can occur.
While crystalline regions add strength to a material, such regions are both rigid and brittle. The amorphous regions, where the chains are in a more random orientation, increase flexibility but decrease the strength of a material. Most commercial polymers have a balance between amorphous and crystalline regions, allowing a balance between flexibility and strength.
Crystallization is favored by the presence of regularly spaced symmetrical units. This is reinforced by the presence of regularly spaced polar groups that can form secondary dipole-dipole interactions. Polymers such as polyamides (nylons), polycarbonates (
17
),
and polyesters tend to form crystalline polymers because of the presence of additional secondary forces. Polymers such as linear polyethylene (with little or no branching) tend to be crystalline because of their symmetry and the ability of their chains to closely approach one another.
Viscoelastic materials
Polymers are viscoelastic materials, meaning they can behave as liquids and solids. When they behave as liquids, their actions can be described by Newton's law, where the applied stress is proportional to the rate of strain. For materials behaving as solids, the mechanical properties can often be described in terms of a spring using Hooke's law, where the applied stress is proportional to the resultant strain. Stress is force per unit area, and strain or elongation is the extension per unit length. See also: Stress and strain
A reinforced or crystallizable elastomer exhibits a relatively low breaking stress but a high elongation. Ductile polymers, such as polyethylene and polypropylene, “give” or “yield,” and at high elongations some strengthening and orientation occur. A brittle polymer, such as polystyrene, does not give much and breaks at a low elongation. A fiber exhibits high strength, high stiffness, and little elongation. In each case, the particular property is dependent on the temperature and the rate in which the stress is applied. Thus, at low temperatures where segmental and whole-chain mobility does not occur, the material can act as a solid. In addition, when a stress is rapidly applied so that chain or segmental mobility is not able to engage, the material will also act as a solid. Thus, a polymer that is brittle or “” at low temperature may become ductile with Newtonian behavior at higher temperatures where segmental or chain mobility can occur (Fig. 4). See also: Elasticity
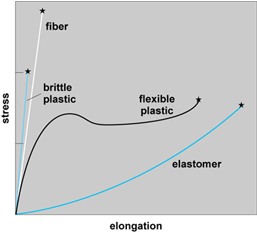
When an applied stress is removed, some or all of the deformation may be recovered, with the material returning to its original shape. The term “creep” is used to describe irreversible deformation. The term “elastic memory” is used to describe situations where a polymer is heated and then deformed and then cooled rapidly enough so that the polymer chains are “frozen” in place, with the material retaining the “deformed” shape. See also: Creep (materials)