Key Concepts
The theory that the universe began in a hot, dense state and has since expanded and cooled. The big bang is the generally accepted cosmological theory for the origin, properties, and evolution of the universe (Fig. 1). See also: Cosmology; Universe
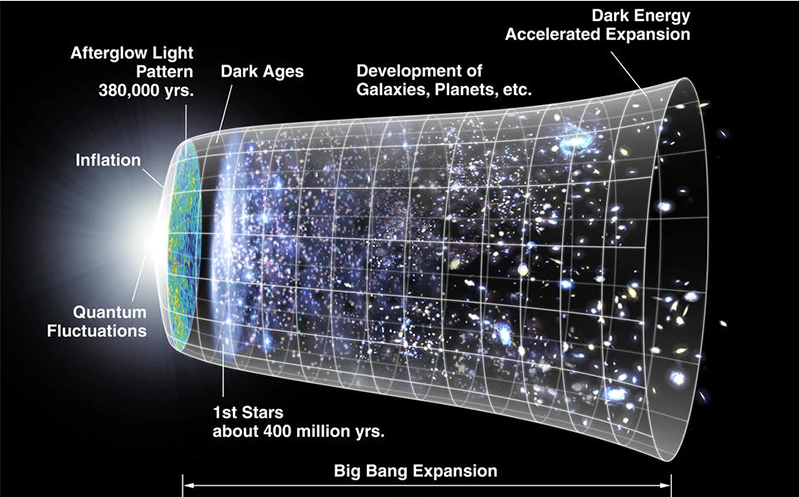
Broadly, the big bang theory describes how the universe began in a hot, dense state that has since expanded and cooled over the past 13.3 to 13.8 billion years, with the calculated age depending on the particular observations and scientific model of the universe that are used. In the first fractions of a second of the big bang's unfolding, the fundamental forces and particles of nature took form. The first atoms of hydrogen and helium then formed about 380,000 years into the universe's history, rendering the universe transparent to particles of light called photons. This light, the "afterglow" of the big bang, now bathes the Earth as a uniform glow of radiation known as the cosmic background radiation. The first stars and galaxies then developed under the influence of gravity approximately several hundred million years post-big bang. Subsequent structure evolution and expansion has given us the universe that we observe back through eons of cosmic history and through present day. See also: Atom; Cosmic background radiation; Elementary particle; Fundamental interactions; Galaxy; Galaxy formation and evolution; Helium; Hydrogen; Nucleosynthesis; Star; Stellar population
This overall narrative of the universe's history, in accordance with the big bang theory, is supported through numerous lines of observational evidence, which include: (1) The universe's expansion, (2) the existence of the cosmic microwave background radiation, (3) the abundances of the lightest chemical elements, in particular hydrogen and helium, and (4) the age of the oldest-known stars. Yet the big bang theory remains an incomplete description of reality, with several problems that have yet to be fully worked out. For instance, versions of the big bang theory incorporating "inflation," which postulates that the universe underwent an exponential increase in size very early in its existence, potentially explain the puzzling uniformity of the temperature of the cosmic microwave background, as well as the universe's geometrical flatness. Another unresolved aspect of the big bang is the cosmic imbalance between matter and antimatter. The big bang models that best match observations also must include the dark matter and dark energy as the dominant constituents of the universe representing about 95% of its composition. Although these substances have yet to be identified, their existence is now widely accepted. See also: Antimatter; Cosmic abundance of elements; Cosmochemistry; Dark energy; Dark matter; Element (chemistry); Periodic table
Theoretical and observational scientists continue to grapple with the big bang theory and its implications. The theory's framework rests upon other bedrock theories in modern physics, namely general relativity and quantum mechanics. The former, the prevailing theory of gravitation originally advanced in Germany by theoretical physicist Albert Einstein in 1916, describes macroscopic reality—on the order of planetary, galactic, and cosmic scales—to extreme precision. The latter theory describes reality on its smallest scales of fundamental particles and three of nature's four forces, conveyed in the standard model of particle physics. See also: Gravitation; Quantum mechanics; Relativity; Standard model
Chronology of the early universe
Modern theoretical work and the union of particle physics with cosmology has traced the universe back through its initial fractions upon fractions of a second of existence (Fig. 2).
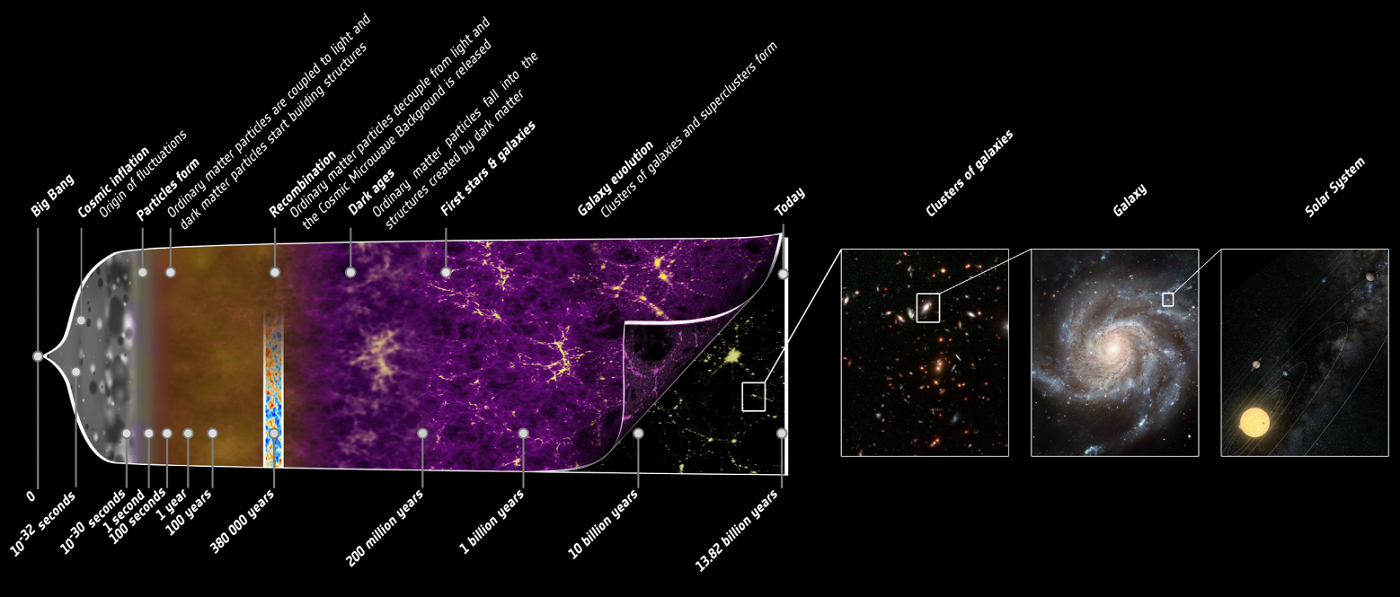
Planck epoch
Present theories cannot go any farther back than about 10-43 s after the initial moment of the universe's origin in the big bang. The immensely hot, dense state of this earliest physically describable universe is referred to as the Planck epoch, after German physicist Max Planck. Before this epoch, Einstein's relativity predicts a singularity of infinite density, representing a breakdown of the theory. Instead, the Planck epoch is thought to involve a quantum-mechanical interpretation of gravitation—quantum gravitation—when all four forces of nature were united, but that “unified theory” has yet to be fully articulated. The temperature of the Planck epoch was a minimum of 1032 K—approximately a trillion trillion billion degrees K, or Celsius, or Fahrenheit, give or take an order of magnitude. See also: Quantum gravitation
Grand unification epoch
At around 10-36 s in the timeline of the big bang, gravitation then split off from the other three fundamental interactions, these being the strong, weak, and electromagnetic. Those three interactions remained theoretically unified in a "grand unification" epoch. Physicists continue to pursue grand unification theories (GUTs) that mathematically convey the conjoining of the three forces at tremendous temperatures around 1029 K and energies around 1016 gigaelectronvolts. GUTs build upon the successful unification of the weak and electromagnetic forces as the electroweak interaction, demonstrated experimentally in the 1970s and 1980s. By about a ten-thousandth of a second later, at 10-32 s, the electroweak interaction first came to exist in distinction from a GUT force, sometimes called an electrostrong force, when the strong interaction separated, a phenomenon known as symmetry breaking in physics. See also: Electromagnetism; Electronvolt; Electroweak interaction; Fundamental interactions; Grand unification theories; Symmetry breaking; Symmetry laws (physics); Weak nuclear interactions
Inflationary epoch
The epoch from 10-33 to 10-32 s is also when many physicists theorize that the universe underwent a sudden and exponential expansion, increasing in size on the order of 1026 times. The cosmological theories describing this transformation of the universe are called inflation. First proposed by U.S. physicist Alan Guth circa 1980 and widely developed thereafter, inflationary universe cosmologies resolve key issues in the big bang theory. The issues include the horizon problem (relating to the uniformity of the cosmic microwave background), the flatness problem, and the magnetic monopole problem (referring to the extreme rarity of hypothetical elementary particles with single magnetic charges). These problems are explained in detail in the "Inflationary universe cosmology" article. See also: Inflationary universe cosmology; Magnetic monopoles
Quark epoch
In the vicinity of 10-12 s, given the temperatures and energies of the early cosmos, much of its contents is in a state known as a quark-gluon plasma. The elementary particles called quarks cannot yet bind via the strong force, carried by gluons, into composite particles called hadrons, which are familiar as the protons and neutrons that comprise atomic nuclei. The most powerful particle accelerator built to date, the Large Hadron Collider at the European Organization for Nuclear Research (CERN), can achieve energies sufficient to return matter briefly to a quark-gluon plasma state. See also: Atomic nucleus; Large Hadron Collider (LHC); Gluons; Neutron; Proton; Quark; Quark-gluon plasma; Strong nuclear interactions
Hadron epoch and antimatter annihilation
By the time the universe was 1 s old, it had cooled enough to allow hadrons to form. Initially in the big bang, equal amounts of matter and antimatter—just like matter, but with opposite properties of charge and quantum number—should theoretically have been created. However, a slight asymmetry between these substances allowed only matter to survive. Neither the big bang theory, nor the standard model of particle physics, can adequately explain the nature of this asymmetry. Some small, yet insufficient asymmetries between matter and antimatter have been discovered to date, described by charge-parity (CP) violation. Researchers continue to experimentally probe antimatter, which is generated through natural processes such as radioactive decay, and its matter counterpart for extensions of asymmetry. See also: CP symmetry and its violation; Electric charge; Quantum numbers
Big bang nucleosynthesis
As the temperature cooled to a billion K (2 billion F), nucleosynthesis began, approximately 10 s after the big bang. The universe's initial hydrogen and helium nuclei formed over the next several minutes during this process. The close matching of measurements of the cosmic ratio of hydrogen to helium to predictions made by big bang theory are a key piece of evidence corroborating the cosmological framework, and measurements of the deuterium (heavy hydrogen) fraction give a value for the density of matter that matches the currently accepted value. See also: Atomic nucleus; Nucleosynthesis
Recombination
Atoms could not form until approximately 378,000 years after the big bang, when the universe cooled to 3000 K (5000°F). Protons and electrons could now combine to make hydrogen atoms, a process called recombination. For the first time, the universe became transparent to particles of light, known as photons. The blackbody radiation of the gas formed at the time of recombination has traveled freely through space ever since, and to our telescopes, as the cosmic background radiation. See also: Blackbody; Heat radiation; Photon
Study of the cosmic microwave background
The discovery of the cosmic microwave background in 1965 was a clinching moment for the general acceptance of big bang cosmology. Observations of its spectrum by the Cosmic Background Explorer (COBE) spacecraft between 1989 and 1993 were in complete agreement with a blackbody curve at 2.728 K (−454.7°F). Scans of the cosmic microwave background by next-generation instruments, namely the Wilkinson Microwave Anisotropy Probe (WMAP) obtained between 2003 and 201, and the Planck mission between 2009 and 2013, provided even more detailed measurements on a finer spatial scale and were in close agreement (Fig. 3). The radiation is very uniform, but with some fluctuations, largely statistical in nature. These fluctuations are thought to have functioned as the primordial "seeds" from which galaxies formed. See also: Cosmic background radiation; Planck mission; Wilkinson Microwave Anisotropy Probe
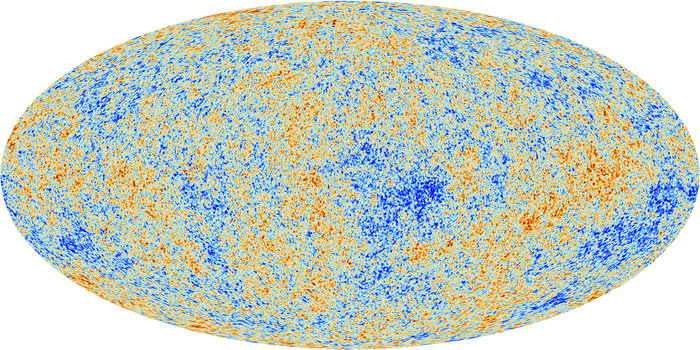
The results from WMAP and Planck have further shown that the universe's content is mostly dark matter and dark energy (Fig. 4). The former is thought to consist of an unknown particle (or particles) that interacts at a minimum with "normal" matter via gravitation but not through electromagnetism. Key evidence for dark matter comes from observing galaxy motions in clusters, galaxy rotation curves, hot gas in elliptical galaxies and clusters, and the phenomenon of gravitational lensing. A comprehensive worldwide search effort remains underway to detect particles of dark matter; candidates have included weakly interacting massive particles (WIMPs) and axions. Dark energy, meanwhile, is a poorly understood driver of accelerating cosmic expansion. A leading candidate for dark energy is a cosmological constant, in essence an inherent energy to the vacuum of space that acts as a negative pressure on cosmological scales. See also: Accelerating universe; Cosmological constant; Dark energy; Dark matter; Gravitational lens; Weakly interacting massive particle (WIMP)
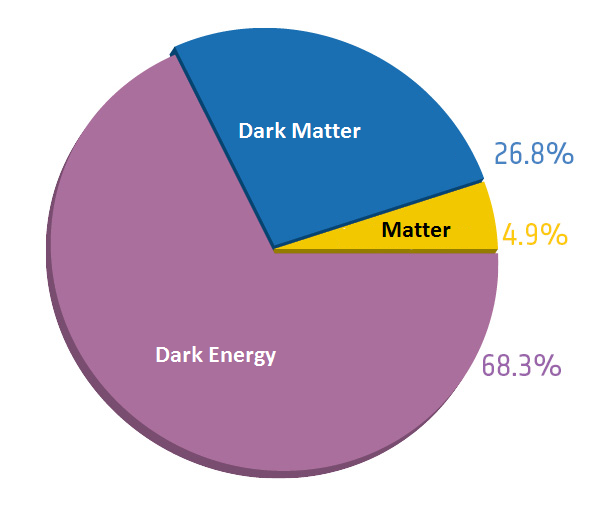
Other evidence for the big bang
Expansion of the universe
In the 1920s, it became clear that the “spiral nebulae,” clouds of gas with arms spiraling outward from a core, observed by astronomers were external galaxies on the scale of the Milky Way Galaxy. U.S. astronomer Edwin Hubble established this fact in 1925 by observing Cepheid variable stars (a special type of variable star for which its period of variation is linked to its intrinsic brightness) in several galaxies, which enabled a determination of the distances to these galaxies with some accuracy. The distances to Cepheid variable stars, and thus the accuracy of the cosmic distance ladder, is being improved by the parallax measurements made from ESA’s Gaia spacecraft. See also: Cepheid variables; Cosmology; Gaia mission; Galaxy; Milky Way Galaxy; Variable star
Years earlier, starting in 1912, the spectra taken of many of these spiral nebulae displayed large redshifts. According to the Doppler effect, these large redshifts correspond to large velocities of recession from the Earth. Estimates of distances to these objects by Hubble and colleagues established a direct relation between the distance to an external galaxy and its velocity of recession. A paradigm of an expanding universe best fit the data. See also: Doppler effect; Redshift; Spectroscopy; Spectrum
An ongoing scientific debate centers on the rate of this expansion, known as the Hubble constant. Observations by the Hubble Space telescope of distant supernovae, along with nearer Cepheid variables, constrains the rate to about 73 (km/s)/Mpc, with an "Mpc" being a megaparsec, or 3.26 million light-years. However, observations of the cosmic microwave background radiation by Planck and WMAP constrain the Hubble constant to 67 (km/s)/Mpc. This discrepancy could point to new physics and significant modifications of the big bang theory. Data from Gaia, as well as an alternative measure of the Hubble constant using gravitational waves, could help settle the debate. See also: Gravitational radiation; Hubble constant; Hubble Space Telescope; Light-year; LIGO (Laser Interferometer Gravitational-wave Observatory); Parsec; Physics; Supernova
Age of stars
The universe must, of course, be older than any of its evolved objects, such as stars. Studies of the Sun and other stars have led to a robust theoretical paradigm for stellar evolution. Plots of stars' luminosity (brightness) and color (temperature), assembled in the Hertzsprung-Russell diagram, for instance, allow astronomers to ascertain the ages and evolutionary state of stars, observed individually or in aggregate in studies of distant galaxies. From these investigations, the oldest stars in the universe have ages exceeding 13 billion years, in accordance with the timeline of the universe's development prescribed by the big bang theory. See also: Hertzsprung-Russell diagram; Star; Stellar evolution; Stellar population; Sun
Cosmic evolution after recombination
According to big bang cosmology, after recombination and the emission of the cosmic microwave background radiation when the universe was 378,000 years old, the universe continued cooling and expanding. The first stars made of primordial hydrogen did not form for tens of millions of years. With no sources of light besides the leftover background radiation and radiating hydrogen, the universe went through an epoch commonly called the Dark Ages.
Over the next several hundred million years, the formation of the first stars and early galaxies, including quasars with actively feeding supermassive black holes in their centers, supplied the radiation that ionized ambient gas throughout the cosmos. This period is known as reionization. See also: Black hole; Galaxy formation and evolution; Quasar; Supermassive black holes
The subsequent 13 billion years have seen multiple generations of stars, galactic growth, and the dominance of dark energy that has now been accelerating cosmic expansion for the past 5 billion years. The Sun and our solar system of planets, asteroids, and comets formed approximately 4.6 billion years ago. See also: Accelerating universe; Asteroid; Comet; Earth; Planet; Solar system
Prospects
The "standard model" of big bang cosmology that has emerged over the last few decades is known as lambda CDM, in which a capital lambda (the letter of the Greek alphabet) denotes dark energy and the CDM stands for cold dark matter, a slow-moving variety of dark matter that best matches observations through simulations. Research continues into detecting and explaining dark matter, dark energy, CP violation, inflation, the true rate of the Hubble constant, and other apparent aspects of our universe through the big bang theory. See also: Cosmology