Key Concepts
Everything in existence, including all matter and energy, and the enormous volume which contains them. The observable universe currently spans about 8.8 × 1023 km (5.5 × 1023 mi), and contains approximately 3.1 × 1054 kg (6.8 × 1054 lb) of matter, yielding an average density equivalent to a few atoms per cubic meter. Most of the universe, then, is empty space; the matter is distributed thinly throughout, forming objects and structures (Fig. 1) at a variety of different sizes. The study of this matter and energy, and its distribution, composition, origin, and evolution, is what constitutes the sciences of astronomy and cosmology. See also: Astronomy; Atom; Cosmology; Density; Energy; Matter
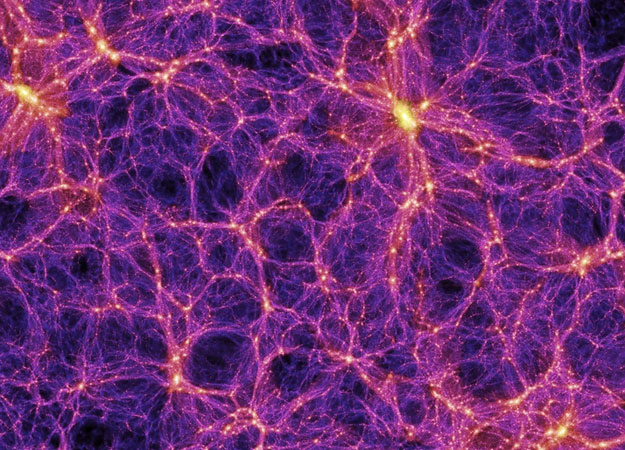
This article will start the cosmic survey with the more familiar physical objects—atoms, planets, stars, and galaxies—following a sequence of increasing size, building up to the largest structures in the universe. A number of lesser-known and less tangible entities, such as energy, exotic particles, and dark matter, will complete the survey. With this synopsis of the constituents of the universe in hand, this article will address the questions of where they all came from and what their ultimate fate will be.
Ordinary units of measure, such as miles, kilometers, pounds, and kilograms, are usually not convenient for dealing with the enormous sizes and masses encountered in astronomy, so astronomers have devised other units. The standard length scale in astronomy is the parsec (pc), equal to 3.1 × 1013 km (1.9 × 1013 mi). For larger distances, kiloparsecs (kpc = 1000 pc) and megaparsecs (Mpc = 1,000,000 pc) are used. The light-year, about one-third of a parsec, often appears in popular astronomy texts, but it is less common at the professional level. The mass of the Sun, 2.0 × 1030 kg (4.4 × 1030 lb), is used as a standard mass unit. Temperatures are measured using the Kelvin scale. A kelvin (K) is the same as a Celsius degree, but the Kelvin scale starts from absolute zero (–273°C) instead of the freezing point of water. To recast the figures quoted above, the observable universe has a diameter of roughly 8400 Mpc, or 93 billion light-years, and contains 4.4 × 1022 solar masses of material. See also: Light-year; Parsec
Baryonic matter
Most of the matter encountered in everyday life is in the form of atoms, or chemically bound groups of atoms called molecules. An atom consists of a positively charged nucleus of protons and neutrons, surrounded by clouds or shells of negatively charged electrons. The protons and neutrons are responsible for most of the mass of the atom. Since both protons and neutrons belong to a class of subatomic particles known as baryons, this ordinary form of atomic matter is called baryonic matter by astronomers. The number of protons in an atom's nucleus determines many of its properties, that is, which of the 118 currently known chemical elements that atom represents. See also: Atomic structure and spectra; Baryon; Chemical element; Elementary particle; Geochemical distribution of elements; Periodic table
A large fraction of the visible matter elsewhere in the universe—planets, stars, nebulae, galaxies—is also baryonic in nature, but the relative proportions of the chemical elements are very different from here on Earth. Hydrogen is by far the most abundant element in the universe, representing 75% of the total baryonic mass. Helium is also plentiful, at about 23% of the total mass. All the heavier elements, collectively referred to as metals (regardless of their solidity or conductivity), make up the remaining 2%, with carbon, nitrogen, oxygen, neon, and iron as the primary constituents. The relative chemical proportions observed on the surface of the Sun (the solar abundances) are used as a reference point for most other abundance measurements (see table). The exact proportions present in any given astronomical object will depend on its age, environment, and formation history. See also: Cosmic abundance of elements
Element | Symbol | Atomic number | Mass fraction | Number fraction |
---|---|---|---|---|
Hydrogen | H | 1 | 0.73 | 0.92 |
Helium | He | 2 | 0.25 | 0.078 |
Oxygen | O | 8 | 0.0077 | 0.00061 |
Carbon | C | 6 | 0.0029 | 0.00030 |
Iron | Fe | 26 | 0.0016 | 0.000037 |
Neon | Ne | 10 | 0.0012 | 0.000077 |
Nitrogen | N | 7 | 0.00095 | 0.000084 |
Silicon | Si | 14 | 0.00069 | 0.000030 |
Magnesium | Mg | 12 | 0.00047 | 0.000024 |
Sulfur | S | 16 | 0.00038 | 0.000015 |
Argon | Ar | 18 | 0.00018 | 0.0000058 |
Nickel | Ni | 28 | 0.000086 | 0.0000018 |
Calcium | Ca | 20 | 0.000058 | 0.0000018 |
Aluminum | Al | 13 | 0.000044 | 0.0000023 |
Sodium | Na | 11 | 0.000030 | 0.0000016 |
Chromium | Cr | 24 | 0.000027 | 0.00000065 |
Chlorine | Cl | 17 | 0.000012 | 0.00000037 |
Manganese | Mn | 25 | 0.000010 | 0.00000023 |
Phosphorus | P | 15 | 0.0000075 | 0.00000030 |
Cobalt | Co | 27 | 0.0000058 | 0.00000012 |
*The proportion of each element is listed both by mass (fraction of the total mass) and by number (fraction of the total number of atoms).
SOURCE: C. W. Allen, Astrophysical Quantities, 3rd ed., Althone Press, 1991.
Stars and stellar evolution
The most numerous components of the nearby universe, as seen in the night sky, are the stars. These pinpricks of light are actually objects much like the Sun, but they appear faint due to their extreme distance from the Earth. Stars are enormous spheres of hot gas (primarily hydrogen and helium), held together by their own gravitation. They are powered by nuclear reactions deep in their interiors, where temperatures and pressures are high enough to fuse hydrogen atoms together into helium, releasing energy in the process. (This process is sometimes referred to as hydrogen burning, although it is quite different from ordinary chemical burning.) The energy generated by this fusion creates an outward force that balances the inward pull of gravity, keeping the star stable at a set radius, or in “hydrostatic equilibrium.” A star's total mass, which may be from roughly 0.08 to 100 times that of the Sun, determines nearly all of its other characteristics, including temperature, luminosity (intrinsic brightness), and how long it shines before running out of fuel. The Sun is an average star, with a surface temperature of about 5800 K, and a projected lifespan of 1010 years. More massive stars have higher core temperatures and pressures, so the fusion reactions take place much faster, and these stars shine brighter and hotter (thus appearing white or blue in color), expending their fuel reserves in only millions of years. Cool, low-mass stars are more frugal with their available core hydrogen, shining dimly but steadily, with a dull red color, for hundreds of billions of years. When plotted on a graph of temperature versus luminosity, this trend appears as a diagonal band known as the main sequence (Fig. 2). See also: Carbon-nitrogen-oxygen cycles; Gravity; Hertzsprung-Russell diagram; Mass-luminosity relation; Nuclear fusion; Proton-proton chain
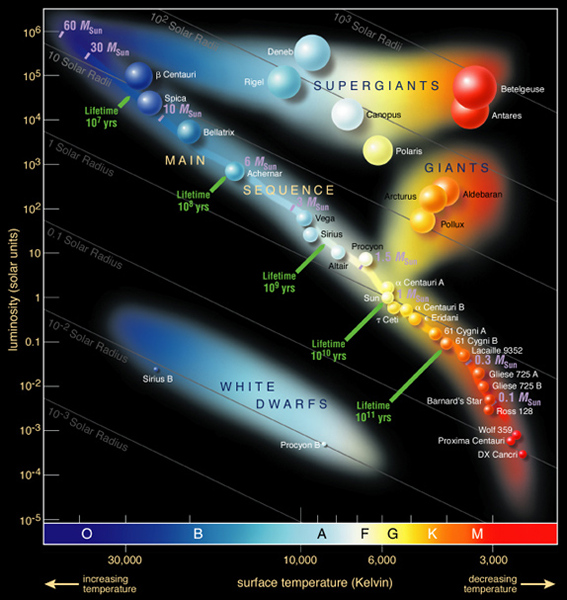
This main-sequence relationship holds only for stars that are burning hydrogen to helium in their cores. Once a star has used up all of its core hydrogen, it must change its internal structure to utilize new fuel sources. First, the hydrogen-burning zone expands outward from the core, and the enhanced power production causes the star to increase significantly in both luminosity and physical size, becoming a red giant or supergiant star. Without the outward pressure of nuclear hydrogen fusion, the core begins to contract gravitationally, compressing the helium gas in this region. Further increases in core temperature and pressure permit the heavier elements to fuse and release energy, but these fuel sources are less efficient and relatively short-lived. A low-mass star (less than a few solar masses) will burn helium for a time, but as the helium fuel is exhausted and the internal furnace wanes, the outer layers of the star are ejected into space, forming a so-called planetary nebula, and the core will gradually shrink into a tiny, dense ember glowing only from its residual heat. This “white dwarf” remnant is supported against further gravitational collapse by electron degeneracy pressure, and will eventually cool and fade as its residual heat is exhausted. See also: Planetary nebula; White dwarf star
Higher-mass stars will burn helium, then carbon, and then a succession of even heavier elements, each for a progressively shorter time, until fusible material of any sort abruptly runs out, and the star collapses catastrophically. Much of the interior mass of the star is compacted into an ultradense core; the outer layers rebound off this core and explode into space, forming a core collapse supernova, which shines for several weeks at a billion times the luminosity of the Sun. Many of the heavier chemical elements are formed in massive stars and during the supernova explosion itself, and are then spread throughout nearby space to be incorporated into future generations of stars. The stellar core is usually left behind as a neutron star, a small, rapidly rotating body consisting almost entirely of neutrons and supported against further gravitational collapse by neutron degeneracy pressure. This neutron star is the densest solid known to exist. Much about the exotic matter in its interior remains unknown. Powerful magnetic fields on the surface of a neutron star can produce electromagnetic waves over a range of energies, which appear to blink on and off as the star spins. A neutron star of this sort is called a pulsar. In the most extreme cases, when the remnant of a massive star left behind after a supernova explosion exceeds roughly three solar masses, the stellar core is compressed so far that it collapses to an infinitesimal point, forming a black hole. Evidence suggests that a resulting, explosive release of energy is so great, it can be seen from across the universe, appearing as a long-type gamma-ray burst (GRB), a relatively brief but exceptionally powerful pulse of high-energy radiation. The dense end states of stars—white dwarfs, neutron stars, and black holes—are collectively termed compact objects. See also: Black hole; Gamma-ray burst; Neutron star; Nucleosynthesis; Pulsar; Star; Stellar evolution
Solar system
Our solar system is thought to have formed from the gravitational collapse of a giant, cool cloud of gas and dust in interstellar space. The Sun is accompanied by a number of smaller objects of various sizes and compositions: eight planets and their satellites, plus a vast reservoir of rocky and icy bodies. The Sun's gravitational domain extends out to almost half a parsec, but its planetary system lies much closer, within about 5 billion km (3 billion mi) of the center. The innermost planets—Mercury, Venus, Earth, and Mars—are small rocky bodies, with solid surfaces and thin atmospheres. Slightly beyond the orbit of Mars is a belt containing several hundred thousand rocky asteroids, fragments of a planet that never quite formed, or perhaps tidally disrupted. Farther from the Sun are the gas giants—Jupiter, Saturn, Uranus, and Neptune—with thick, turbulent, gaseous atmospheres surrounding small liquid or solid metallic cores. Beyond the orbit of Neptune at distances of over 6 billion km (4 billion mi) lies a vast expanse of icy bodies known as Kuiper Belt Objects (KBOs). Over 2500 of these KBOs are currently known. Even farther out lies the Oort Cloud, a spherical shell containing yet more balls of ice, some of which occasionally plummet toward the inner solar system, get heated by the energy of the Sun, and appear as comets, as do some of the KBOs. See also: Asteroid; Comet; Kuiper belt; Planet; Solar system
Exoplanets
In the 1990s, astronomers first learned that the Sun is not the only star with a planetary system. Thousands of exoplanets are now known, with most having been discovered via the transit technique by the Kepler mission. See also: Exoplanet; Kepler mission; Transit (astronomy)
Interstellar material
The “empty” space between the stars actually contains significant amounts of matter—some of it distributed continuously, some of it concentrated in enormous dark clouds—known collectively as the interstellar medium. An atomic hydrogen component is distributed fairly smoothly, although with some variation in temperature and density. Also present are tiny solid dust particles, typically with diameters of 0.00025 cm (0.0001 in.) and smaller, and composed of graphite, silicon oxide, and complex carbon-chain and carbon-ring molecules. See also: Interstellar matter
Also scattered throughout space are denser clouds of molecular hydrogen (H2), carbon monoxide (CO), and more complex molecules, sprinkled with the heavier elements. Some of these clouds, or dark nebulae, are many parsecs in size, and contain enough raw material to form a million stars like the Sun. In fact, these clouds often are the sites of star formation, when external gravitational disturbances or shock waves trigger the collapse of portions of the clouds. The densest clumps of mass within the cloud, having a stronger gravitational pull, will accumulate more of the surrounding material, increasing the pressure and temperature in the core of the clump to the point where hydrogen fusion can start. Star-forming regions, viewed with instruments such as the Hubble Space Telescope (Fig. 3), are some of the most beautiful phenomena in the nearby universe, as new stars emerge from the residual gas surrounding their birthplaces. See also: Hubble Space Telescope; Molecular cloud; Protostar
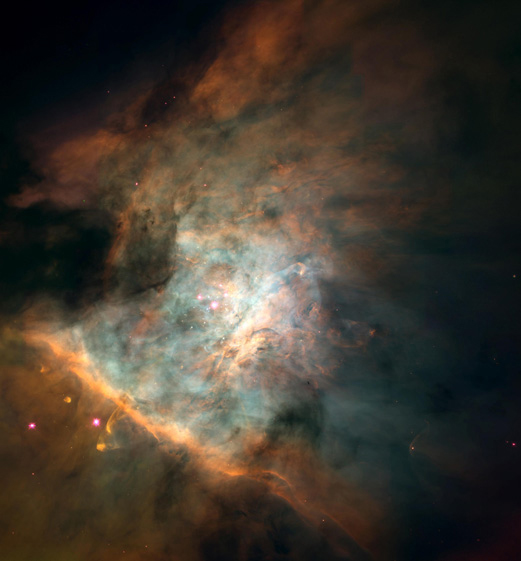
Sometimes these clouds form self-gravitating spheres of gas which do not have the mass of approximately 0.08 solar mass that is required to support sustained internal fusion, and thus will not shine like stars. Nonetheless, these objects still glow dimly from the residual heat of their gravitational collapse. These “failed stars” are called brown dwarfs, because they are very dim and red compared to real stars. See also: Brown dwarf
Interstellar space is also populated by high-velocity protons, electrons, and other particles which have been accelerated by supernova explosions and other mechanisms to nearly the speed of light. These energetic particles are called cosmic rays. A cosmic-ray proton, despite being just a single subatomic particle, can possess the equivalent momentum of a fast baseball. Most cosmic rays originate within the Milky Way, but some may have reached Earth from other galaxies. See also: Cosmic ray
Galaxies
Stars and interstellar matter are not distributed uniformly throughout the universe, but cluster together in vast units known as galaxies, each containing roughly 10 million to a trillion stars. These galaxies can range over many orders of magnitude in brightness, though few can be seen from Earth with the naked eye. Many thousands of galaxies can be imaged with telescopes, even in patches of sky thought to be “empty” in previous decades. It is now estimated that galaxies number in the hundreds of billions throughout the universe (Fig. 4).
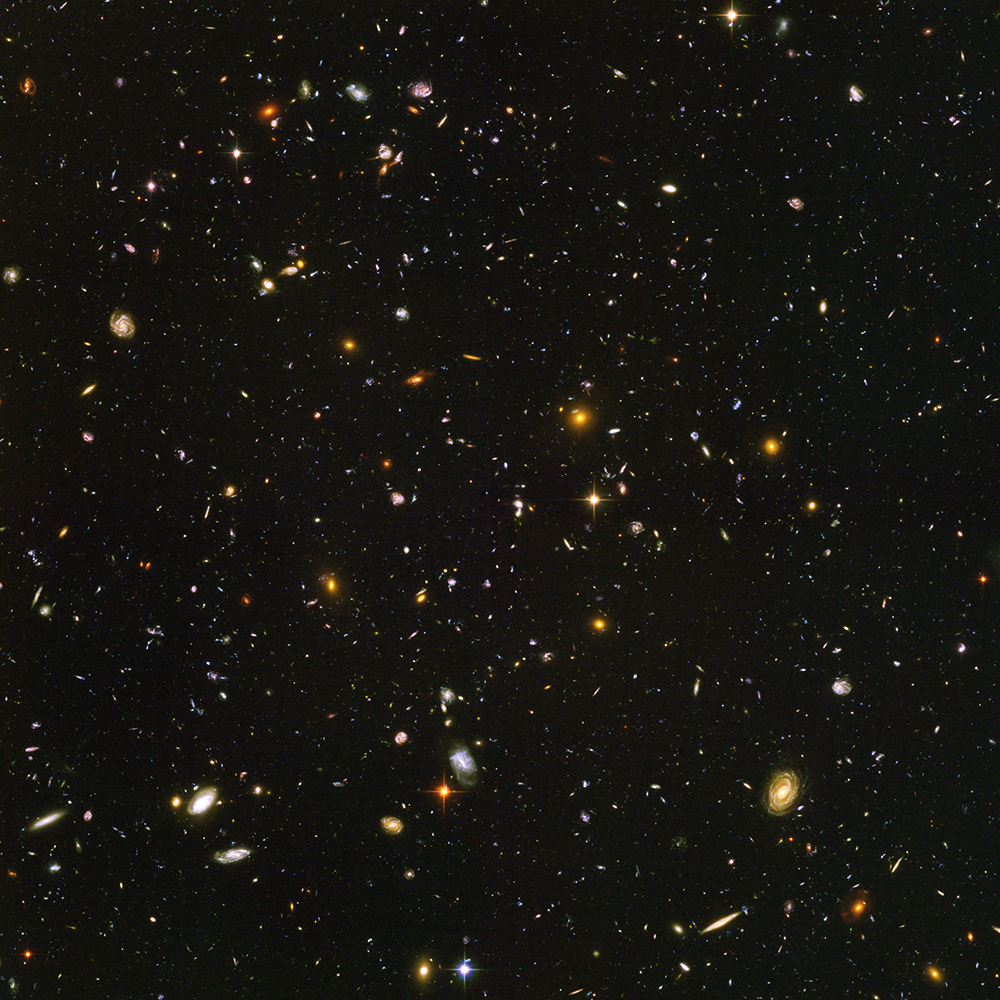
Galaxies are categorized into three types—spirals, ellipticals, and irregulars—with numerous subclasses based on size and structure.
The Milky Way, the galaxy containing our solar system, is a typical spiral galaxy—a flattened disk of some 300 billion stars (Fig. 5a). An oblate central bulge, consisting mostly of older red stars, occupies the central region of the galaxy. The bulge is surrounded by a thin disk of younger stars. Within the disk, a pattern of spiral arms is defined by bright blue stars, recently formed from the gas clouds which also inhabit the disk. Both the bulge and the disk are embedded in the halo, a sparsely populated sphere of very old stars, some of which date back to the formation of the galaxy. The Milky Way, like many other spirals, is accompanied by an assortment of dwarf galaxies and globular clusters (small, tight collections of only thousands or millions of stars), which orbit the main galaxy. Dwarf galaxies that approach too closely to the galactic center are ripped apart by the galaxy's gravitational field, and their component stars are absorbed into the halo, suggesting that other such mergers in the past may explain the current size and stellar composition of the Milky Way. The visible portion of the disk extends about 30 kpc from center to edge, although the orbital motions of the stars and gas strongly imply a significant component of nonluminous, so-called dark matter both within the visible galaxy and beyond. Other spiral galaxies also exhibit this characteristic, implying that dark matter is a common constituent of galaxies. See also: Dark matter; Milky Way Galaxy; Star clusters
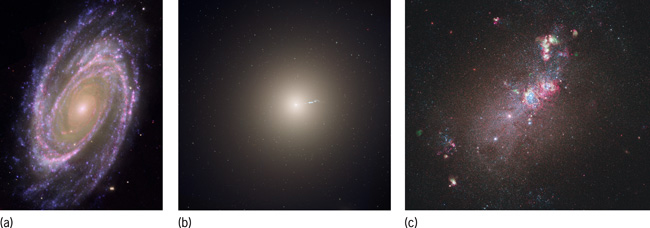
Elliptical galaxies are simpler systems than the spirals, consisting mostly of a single population of cool, low-mass stars, arrayed in a spherical or oblate configuration (Fig. 5b). Ellipticals have already converted almost all of their interstellar matter into stars, so that only the long-lived red stars now remain; young blue stars, like those found in spiral arms, have long since run out of fuel and expired. Ellipticals span a much wider range in size than the spirals: Dwarf ellipticals, such as those orbiting nearby spiral galaxies, may contain only a few million stars, while the giant ellipticals that inhabit the centers of major clusters have upward of a trillion stars. As in spirals, the stars in ellipticals orbit the center of those galaxies at speeds which require significant additional dark mass, beyond that evident in the form of stars, to keep the galaxy from flying apart. Ellipticals are more likely than spirals to be found within galaxy clusters, which suggests that collisions or interactions between galaxies play some role in the formation of the elliptical type.
The irregular type includes all galaxies not readily recognizable as spirals or ellipticals (Fig. 5c). Irregular galaxies are similar to spirals in that they often exhibit active star formation, but they do not share the well-ordered structure of spirals. They may be the result of violent galaxy collisions, or perhaps are smaller galaxies that were prevented from developing structure due to gravitational disruption from a nearby larger galaxy. The Milky Way's two largest companions, the Large and Small Magellanic Clouds, are irregulars. See also: Galaxy; Galaxy formation and evolution; Magellanic clouds
Quasars
Supermassive black holes, similar to the stellar-sized black holes which form during the supernova explosion of a massive star but containing millions or billions of solar masses instead of only a few, exist at the centers of many galaxies, including the Milky Way. Black holes themselves cannot, by definition, emit any light or other radiation, but they can serve as highly efficient engines for converting mass into energy. As stellar or interstellar matter is drawn into the black hole, it forms an accretion disk around the central point and is heated by friction to temperatures of hundreds of thousands of kelvins, so that it glows brightly with a luminosity equivalent to trillions of Suns. At the far reaches of the universe, these galaxy cores appear as faint starlike points of light, and were thus labeled quasi-stellar objects or quasars when they were first discovered. Nearby galaxies such as the Milky Way do not appear as quasars, because the flow of fuel to the black hole has slowed to a trickle, and the central engine shines only dimly. Farther away, however, the ultraluminous galaxy cores are seen as they were many billions of years ago, when the central engines were still being supplied with copious fuel. See also: Quasar
Groups, clusters, and large-scale structure
Galaxies themselves are usually bound together in groups (up to about 50 galaxies) and clusters (50 to thousands of galaxies) spanning regions 2–10 Mpc in diameter (Fig. 6). Like individual galaxies, groups and clusters are gravitationally self-contained systems, only on a larger scale. Clusters must contain more mass than is immediately evident—the total mass of the visible galaxies in a typical cluster is only a small fraction of the amount needed to keep the fast-moving galaxies from flying out of the cluster. There must therefore be some other mass component in addition to the galaxies, contributing its gravitational potential to keep the cluster together. Some of this “missing mass” can be attributed to hot diffuse gas, the intracluster medium, which is visible to x-ray and ultraviolet telescopes and fills the space between cluster galaxies, but the nature of the rest of the dark matter is yet to be explained. See also: Local Group; Virgo Cluster
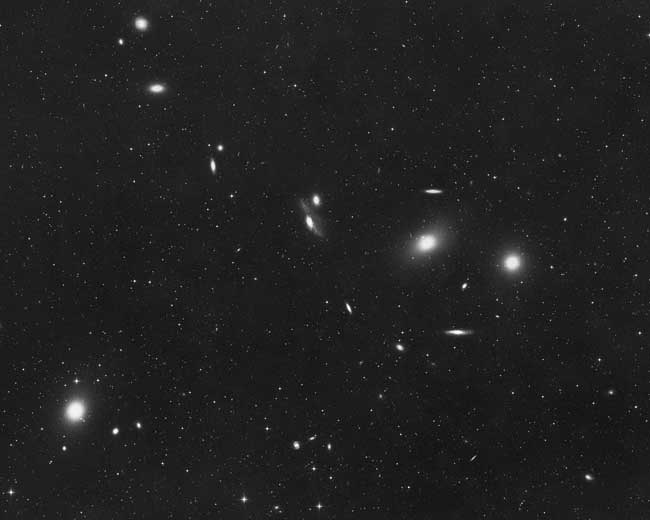
Clusters accumulate into yet larger entities called superclusters. Typical superclusters are of the order of 100 Mpc in size, and often take elongated or filamentary shapes. Redshift surveys, astronomical programs to measure the velocities of thousands or millions of distant galaxies and thus estimate their distances, have provided glimpses of these largest known levels of structure in the universe (Fig. 7). Groups and clusters seem to be concentrated in thin sheets, surrounding enormous voids with very few galaxies. Superclusters sit at the edges and vertices where surfaces intersect. The arrangement has been likened to a froth of soap bubbles, and may provide vital clues to the mechanisms by which structure formed out of the nearly homogeneous early universe.
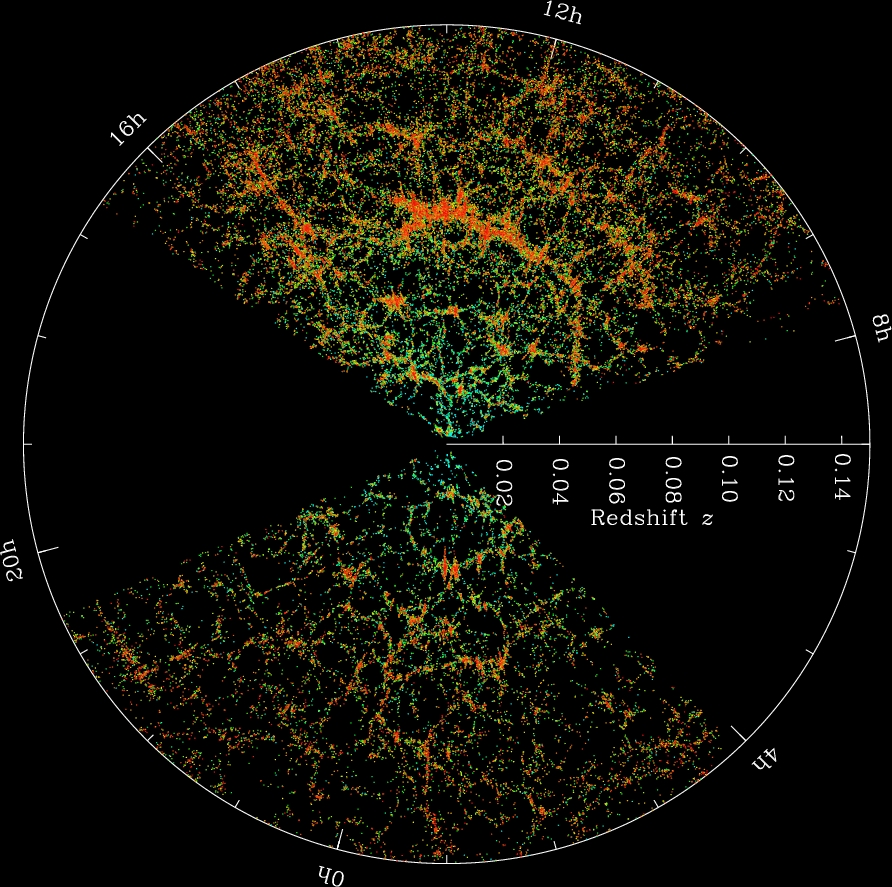
Antimatter (antibaryonic matter)
All subatomic particles have oppositely charged antiparticle counterparts. When a particle and its corresponding antiparticle collide, they annihilate, converting all their mass into energy. Both matter and antimatter are expected to have been formed in the early universe, but clearly not in precisely equal amounts, because the observable universe is utterly dominated by matter. If slightly more matter were formed than antimatter, as predicted by current theory, then later matter–antimatter annihilation would leave behind only the “extra” matter, as observed today. Trace amounts of antimatter are still found in some astronomical environments, where new particle–antiparticle pairs are created out of free energy, in the opposite reaction from annihilation. Collisions between cosmic rays or high-energy photons can supply such energy, creating electrons and their antiparticles, positrons. See also: Antimatter; Electron-positron pair production and annihilation
Nonbaryonic particles
Although most observable matter is baryonic in nature, several kinds of nonbaryonic matter also exist in the universe. Some nonbaryonic particles have been observed experimentally, and play a crucial role in explaining many processes, from nuclear fusion to supernova explosions. Other nonbaryonic particle types have been proposed on the basis of theory but have not yet been detected directly. According to current astronomical measurements, nonbaryonic matter accounts for approximately 23% of the total density of the universe, so it is important to determine the identity and characteristics of this component of the universe.
Electrons, strictly speaking, are not baryons, but they are considered as an integral part of ordinary atomic matter, and thus are usually not categorized with the more exotic nonbaryonic species described below. The net electric charge of the universe is assumed to be zero, so there are the same number of electrons as protons, but because the proton mass is almost 2000 times that of the electron, electrons make only a small contribution to the total cosmic mass. See also: Electron
A large number of other nonbaryonic particles, including mesons, muons, and taus, have been discovered in particle physics experiments. Many of these particles are quite massive (by subatomic standards), but they also have very short lifetimes—they decay rapidly into less massive particles. As such, they are considered unimportant for cosmology.
Neutrinos are electrically neutral, very-low-mass particles that are generated in nuclear reactions. They were once thought to be completely massless entities, but theoretical and experimental evidence now suggests that they have a tiny but nonzero mass, approximately 2 × 10−34 g (4 × 10−37 lb). Neutrinos do not interact strongly with baryons; they pass right through most ordinary matter, and even stars and planets are virtually transparent to them. This characteristic makes them useful tools for studying otherwise inaccessible environments, such as the fusion core of the Sun or the centers of supernovae, but it also makes neutrinos difficult to detect and measure, as they usually pass through any sort of detection device without leaving a trace. Instruments that detect neutrinos from the Sun and other astronomical sources offer a unique means of studying the universe. See also: IceCube Neutrino Observatory; Neutrino; Neutrino astronomy; Solar neutrino
According to new theories of particle physics, a variety of other nonbaryonic particle species might also exist. Some of these hypothetical particles could be much heavier and slower than neutrinos, but like neutrinos, would only interact with baryonic matter via the gravitational force. The most likely candidate to fill this role is the so-called weakly interacting massive particle (WIMP). Lightweight axions are another possibility, along with magnetic monopoles. Experiments attempting to detect WIMPs and other particles directly have not yet proven the existence of any of these exotic entities. See also: Magnetic monopole; Weakly interacting massive particle (WIMP)
Dark matter
A large fraction of the mass of the universe is in some form that cannot be seen but that is evident from its gravitational effect on the motions of bright objects, such as stars and galaxies. This dark matter, or “missing mass,” is present on many different scales, from galaxies to the universe as a whole. Several possibilities for the nature of the dark matter have been suggested. Numerous small, dim baryonic objects, such as faint red stars, brown dwarfs, or black holes, located in the halos of galaxies, might account for some of the galactic-scale dark matter. However, searches for these MACHOs (massive compact halo objects) have turned up far fewer confirmed detections than would be necessary to explain all the missing mass. Furthermore, several independent lines of argument indicate that baryons can comprise only about 4% of the total mass–energy density of the universe, while the dark matter has to make up approximately 23% of the cosmic total in order to properly explain the distribution and motion of galaxies. Dark matter must therefore consist primarily of nonbaryonic particles. Neutrinos were once considered as likely candidates, but observations of the cosmic microwave background argue against this possibility, because neutrinos travel at nearly the speed of light and would spread out too quickly to permit the observed lumpiness of matter in the early universe. Slower-moving (“cold”) particles such as WIMPs have therefore been favored. Active theoretical and experimental efforts are underway to determine the characteristics of such particles, and the effect they would have on the distribution and dynamics of the luminous matter, but direct detection of these particle species will be very challenging. The identification of dark matter remains one of the key unresolved issues of modern astrophysics. See also: Cosmic background radiation; Dark matter
Energy
Energy is a physical entity as real as matter, but it is less tangible, which makes it more difficult to categorize easily. Like matter, energy comes in many different forms, which can be readily transformed from one to another. Energy can also be converted to and from matter. Various forms of energy that play a significant role in the universe—gravitational, electromagnetic, kinetic, nuclear, and dark energy—are described below. See also: Energy
Gravity is the predominant physical force in most astronomical contexts, and is thus responsible for the existence of most of the universe's structure, including stars, planets, galaxies, clusters, and superclusters. Gravitational potential also drives many of the key energy-generating mechanisms in the cosmos, such as nuclear fusion in stellar cores and accretion disks onto black holes in quasars. See also: Gravity
Energy is readily transmitted throughout the universe via electromagnetic radiation, which may take the form of visible light, radio waves, x-rays, gamma rays, or any other part of the electromagnetic spectrum. Electromagnetic energy may be described either as waves—intertwined electric and magnetic fields rippling through empty space—or as particles called photons, which travel through space along null geodesics (that is, straight lines in empty space, or curved lines in the presence of a large mass). The universe now contains about 400 photons per cubic centimeter (6500 per cubic inch), so photons outnumber atoms by a large margin. See also: Electromagnetic radiation; Light; Photon; Relativity
Energy may also be transmitted as gravitational radiation. Gravitational radiation was originally predicted by German-born U.S. theoretical physicist Albert Einstein and propagates through space under the same basic principles as electromagnetic waves; however, rather than resulting from intertwined electric and magnetic fields, gravitational waves are produced by the intertwined motions of very massive objects that are very close to each other. The inspiral and merger of two black holes or other compact stellar remnants produce copious amounts of gravitational waves. The gravity of these massive, dense objects warps the space around them, causing compressions and rarefactions in the fabric of spacetime. This warp grows larger and propagates outward as they orbit each other, much as ripples on the surface of a pond propagate outward when a pebble is dropped in the water. Gravitational wave detectors such as the Laser Interferometer Gravitational-wave Observatory (LIGO) have become operational around the world. The first direct gravitational wave detection was registered by LIGO in 2015, which opened up a new window through which to view the universe. See also: Gravitational radiation; LIGO (Laser Interferometer Gravitational-wave Observatory)
Matter may contain energy (in addition to the rest-mass energy defined by relativity) by virtue of its motion. This kinetic energy may be due to large-scale bulk motion, such as a planet hurtling through space, or it may be in the form of thermal energy—the microscopic motion of an object's constituent atoms, vibrating or moving relative to each other. The higher the temperature, the faster is the average motion of the atoms, and the more energy is present. See also: Kinetic theory of matter
Large reserves of energy are stored in the force that holds atomic nuclei together. There are actually two such forces, the strong nuclear force and the weak nuclear force, which play different roles at the subatomic level. Although the weak nuclear force is considerably weaker than the strong force, the weak force plays an important role in radioactive decay and the formation of isotopes of the heavy elements. The strong force maintains the nuclear structure on which matter is based, but some of this energy can be released in other forms during nuclear reactions. When two hydrogen atoms are fused together to form helium, for instance, the hydrogen nuclei have a greater total binding energy than the resulting helium nucleus, and the difference is released as free energy (in the form of photons and neutrinos). Energy release can also take place when a large, heavy nucleus splits into two lighter nuclei with less net energy. This is the mechanism behind nuclear fission, as found in nuclear power plants and uranium/plutonium bombs. However, because of the relative scarcity of heavy fissionable elements in the universe, fission does not play any appreciable role in astronomical environments. See also: Atomic bomb; Nuclear fission; Nuclear power; Nuclear reaction; Radioactivity; Strong nuclear interactions; Weak nuclear interactions
Research suggests that, on cosmological scales, gravitational attraction is countered by a previously undetected form of energy, known as dark energy or the cosmological constant, which causes space to expand. A repulsive force of this sort had originally been suggested by Einstein's equations of general relativity, but the strength of this force was assumed to be zero, because no excess expansion rate had been observed in the motions of galaxies. Since 1998, however, observations of distant supernovae and the cosmic background radiation have led astronomers to the conclusion that the expansion of the universe is indeed accelerating, and that dark energy is, in fact, the dominant component of the universe. According to these data, dark energy comprises 73% of the total density of mass and energy in the universe, “outweighing” the dark matter and baryonic matter by a factor of three. The actual nature of dark energy is still a mystery. See also: Cosmological constant; Dark energy; Relativity
The traditional four basic forces of nature—gravity, electromagnetism, and the strong and weak nuclear forces—may in fact all be different facets of a single fundamental force. Particle physics experiments show that under conditions of extremely high energy, the weak nuclear force and electromagnetism merge into a single “electroweak” force. Theoretical efforts are being made to devise a grand unified theory under which the strong force is also incorporated, and a still more general force that also includes gravity. Such a unified force may have existed during the moments following the big bang, and then fragmented into separate forces as the universe expanded and cooled. With the discovery of the long-range “antigravity” effect of dark energy, the task of combining all of the known physical forces into a single coherent framework has become even more challenging. See also: Electroweak interaction; Fundamental interactions; Grand unification theories; Standard model; Supersymmetry
Origin, evolution, and fate
The universe is a dynamically evolving system; there are compelling reasons to believe that it has not always been in its current state. Most of the evidence for this assertion is indirect, as humans have not observed the cosmos for long enough to actually see things change on large scales. However, by closely studying the current distribution and motion of matter and energy, as well as collecting the “fossil light” from distant objects, scientists have constructed a consistent picture of the creation of the universe, known as the big bang theory, which explains the observations fairly well. Many of the specifics are still unresolved, however, and are currently the subject of research and debate.
Big bang
The observable universe originated approximately 13.8 billion years ago in a fiery cataclysm termed the big bang. This was not an explosion of compressed matter and energy into a previously empty space. Instead, space itself, as well as everything contained therein, sprang from a single point of near-infinite density and temperature, growing to the volume observed today. As the universe expanded, it cooled, allowing familiar forms of matter to condense from the high-energy “soup” of energy and subatomic particles that constituted the very early universe. The framework for the present-day distribution and dynamics of matter in the universe was also established at these very early times, and thus remnants of the extreme conditions that existed at the beginning can still be seen. Much of the current understanding of the big bang and the early universe hinges on two key observations: velocities of distant galaxies, and the cosmic background radiation. See also: Big bang theory
Cosmological redshifts
The relative velocity of an astronomical object, toward or away from an observer, can be determined very precisely by measuring the color distribution of the light from the object. Distinct spectral features, characteristic of the different chemical elements that are present, appear at well-established locations in the spectrum, but the entire spectrum is shifted toward shorter wavelengths (toward blue) for an object approaching the observer, or toward longer wavelengths (toward red) for a receding object. The amount of this Doppler shift is closely related to the velocity of the object relative to the observer. See also: Doppler effect
If galaxies were moving randomly in the universe, observers would expect to see just as many blueshifted galaxies as redshifted galaxies, roughly half moving toward Earth and half moving away. However, measurements of galaxy motions made in the early twentieth century showed a very different distribution: With the exception of a few nearby galaxies, all galaxies exhibited redshifts of varying amounts, implying that they were all moving away from Earth. U.S. astronomer Edwin Hubble extended this work by determining the distances to these galaxies, finding that recession velocity was directly proportional to distance—the more distant galaxies were moving away faster (Fig. 8). The constant of proportionality relating speed and distance now bears Hubble's name. An increasingly well-supported and accurate determination of this Hubble constant (H0) has been one of the central pursuits of modern astronomy for the past several decades.
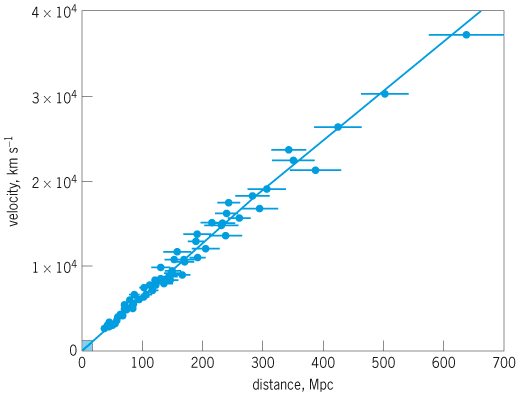
The observation that nearly all galaxies are moving away from Earth's location in space might suggest that Earth is at a special location in the universe, at or near the center of an expanding distribution of matter. It is highly unlikely, however, that Earth would happen to be located at such a special position. Fortunately, these observations can be explained in another fashion. If space itself is expanding uniformly in all directions, and the galaxies are being carried along in this general expansion, then any point in the universe would see all other points moving away. The greater the distance between two points, the more space exists between them, and the faster this distance increases. Hubble's law is therefore a consequence of the uniform expansion of space, which causes more distant galaxies to exhibit larger redshifts because they are receding from the observer faster.
If space is expanding uniformly in all directions, then the universe was smaller in the past. All the matter and energy that is observed in the current-day cosmos would have been present in this smaller volume, so the density (average mass per volume) and temperature (related to the average energy per volume) of the universe would have been higher than they are today. At even earlier times, the volume would have been yet smaller, and the density and temperature even higher, and at some point in the past all matter and energy may have existed in a single point of near-infinite temperature and density. See also: Hubble constant; Redshift
Cosmic background radiation
If this picture is correct, and the current universe was spawned from just such a primordial fireball, then the sky should be filled with a residual afterglow from the era when the matter in the universe was hot and emitting strongly, in much the same way that the surfaces of stars shine today. This primordial radiation will have cooled dramatically, though, because the space containing it has expanded since the radiation was emitted. From its initial characteristic temperature of about 3000 K, this cosmic background radiation, or cosmic microwave background, is expected to have cooled to about 3 K above absolute zero, so that instead of existing mostly as visible light, the energy distribution will have shifted far to the red, into the microwave region of the spectrum.
This microwave background was first detected by German-born U.S. radio engineer Arno Penzias and U.S. radio astronomer Robert Wilson in 1965 as persistent low-intensity static in microwave communications equipment, identified as being of cosmic origin. Subsequent ground-based measurements of the energy distribution of the cosmic background radiation suggested a characteristic temperature of approximately 3 K, but it was not until the Cosmic Background Explorer (COBE) satellite mission in 1990 that the spectrum was measured simultaneously over a wide range of wavelengths, firmly establishing the cosmic background radiation temperature at 2.726 K.
Because the cosmic background radiation is an intrinsic characteristic of the universe, observers expect to see it reaching the Earth uniformly from all directions in space, and the COBE measurements confirmed that this is indeed the case, once the Doppler effect from the Earth's motion through space is subtracted. Subsequent observations of the cosmic background radiation by highly sensitive microwave telescopes, including the Wilkinson Microwave Anisotropy Probe (WMAP) and the Planck spacecraft, have revealed faint ripples in the temperature distribution of the background radiation (Fig. 9). These patterns in the cosmic background radiation are a result of the very first clumps of matter that accreted in the early universe, as regions with slightly different densities emitted slightly hotter or cooler radiation. By measuring the amplitude and angular size of these temperature variations, researchers have been able to deduce the age, total density, and early history of the universe with unprecedented precision. See also: Cosmic background radiation; Planck mission; Wilkinson microwave anisotropy probe
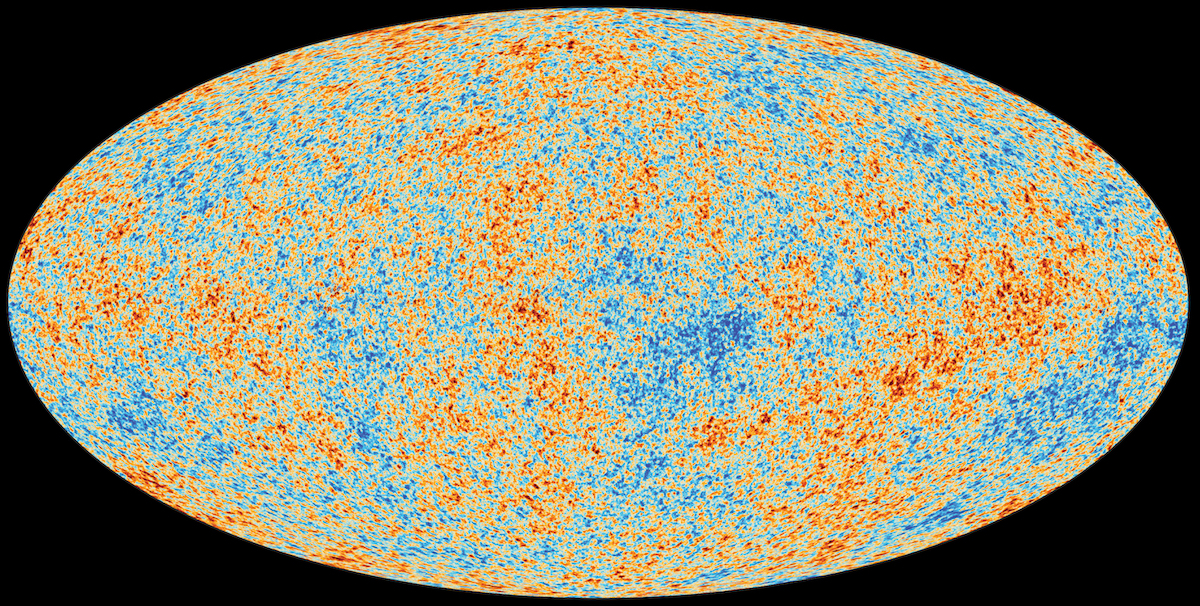
Evolution of the universe
The best models for the beginning of the universe start at 10−43 s after the big bang itself. The conditions before this point were so extreme that the current understanding of physics is insufficient to say anything meaningful (although new theories are being developed to address this limitation). At 10−42 s, the universe was 1045 times smaller than it is today (approximately 6 × 10−18 cm, or 2 × 10−18 in.), and had a mean temperature of 1030 K. At such temperatures, matter as presently known cannot exist, because the energies are so enormous that even the protons and neutrons themselves are torn apart into separate sub-subatomic particles called quarks. The universe was a featureless mixture of subatomic particles and high-energy photons. See also: Quark
As the universe expanded and cooled, however, more particles were permitted to form, once they could exist without being immediately destroyed. At 10−34 s, when the temperature had dropped to 3 × 1026 K, heavier exotic particles such as magnetic monopoles could have emerged. Around this time, the rapidly enlarging structure of space may have undergone an era of even faster expansion, driven by the energy of space itself. This era of hyperfast inflation helps to explain features of the present-day observable universe, such as the uniformity of the cosmic background radiation over the entire sky, and the fact that the average mass density of the universe is high enough for structures such as stars and galaxies to form, but not so large that it would immediately recollapse upon itself. See also: Inflationary universe cosmology
When the temperature had dropped further, to about 3 × 1012 K, protons and neutrons condensed out of the quark mixture. It was still much too hot for electrons to bind to nuclei and form atoms, but more complex atomic nuclei—deuterium (proton plus a neutron), helium-3 (2 protons plus a neutron), helium-4 (2 protons plus 2 neutrons), and lithium (3 protons plus 4 neutrons)—were created, in a fashion similar to stellar core fusion. This era of big bang nucleosynthesis established the initial composition of the universe, about three-quarters hydrogen, one-quarter helium, and a smattering of lithium, from which all subsequent stellar and supernova nucleosynthesis has proceeded.
Temperatures were still too high for electrons to join these nuclei to make complete atoms, until a time about 380,000 years after the big bang. Up to this point, the universe was relatively opaque, because unattached electrons are very good at absorbing light and other electromagnetic radiation. Once the temperature fell below 3000 K, however, protons and electrons could combine into neutral hydrogen atoms, and the universe suddenly became transparent to most wavelengths. The cosmic background radiation formed at this point, and has had little interaction with matter since, which is why observations with microwave telescopes such as Planck reveal such detailed information about this epoch.
The formation of structure—the development of clumpiness in the universe, which appears today as stars, galaxies, and clusters—started slightly prior to matter–radiation decoupling. These very first clumps of mass left their faint imprint on the temperature distribution of the cosmic background radiation, and then continued to accumulate and grow after becoming independent from the radiation field. The exact sequence of structure formation—whether many smaller objects such as stars formed first and then accumulated to form galaxies and clusters, or whether matter clumped on galaxy- or cluster-sized scales and then subdivided into smaller bodies—is still not well understood, because of the scarcity of luminous objects and the difficulty of detecting them at such extreme distances. Sophisticated computer simulations, combined with indirect evidence from observations of the cosmic background radiation and the most distant quasars, suggest that massive stars (100 times the mass of the Sun, or greater) formed relatively early and then exploded as supernovae, pumping energy and newly formed heavier elements into the surrounding clouds of hydrogen and helium, thus setting the stage for the formation of galaxies and stars observed today. Observations from future generations of space telescopes will help resolve many of the uncertainties regarding this era of cosmic history. See also: Supermassive star
Eventually, within a billion years of the big bang, larger structures such as galaxies did take shape, and within them subsequent generations of stars coalesced from interstellar clouds of hydrogen. The Sun (along with its system of planets) appeared about 5 billion years ago. In elliptical galaxies, nearly all the gas has been turned into stars by now, while spiral and irregular galaxies continue to spawn new stars at a steady rate. Large galaxies gradually absorb and consume any dwarf galaxy companions that approach too closely, and occasionally two large galaxies will collide and merge. Meanwhile, the overall expansion of the universe continues (Fig. 10).
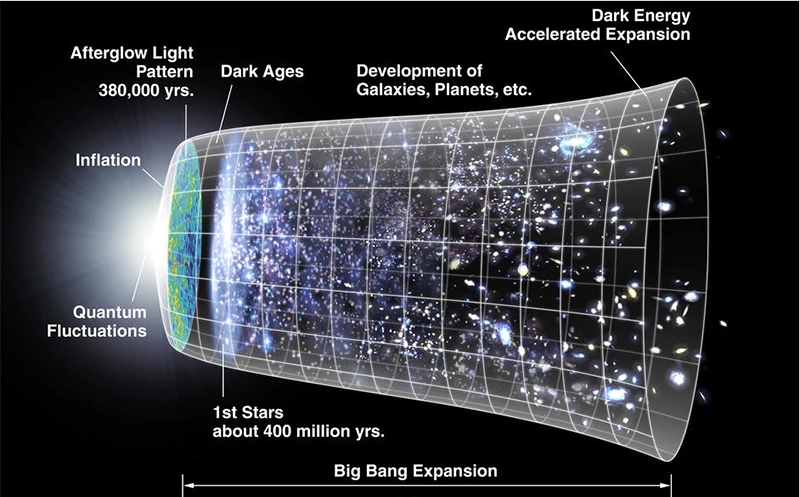
Ultimate fate of the universe
After another roughly 5 billion years, the Sun will run out of hydrogen fuel in its core, and after swelling up into a red giant, will collapse into a white dwarf, gradually fading in brightness as it cools. Most of the other stars that are seen in the night sky will meet a similar fate, some of them within a few million years, others lasting hundreds of billions of years before their fuel reserves run out. New generations of stars will be formed to take their place, but over time the interstellar hydrogen reserves of spiral galaxies such as the Milky Way will be exhausted, and the formation of new stars will cease, leaving only the long-lived dim red stars and stellar remnants such as black holes, neutron stars, and white dwarfs.
On larger scales, the universe will probably continue to expand indefinitely. It was once thought that if the average cosmic density of matter were high enough, the mutual gravitational attraction would be sufficiently strong to gradually slow the expansion to a standstill, and then cause the universe to contract, eventually collapsing in a “big crunch.” According to the current tally of baryonic and dark matter, however, there is not enough gravitational mass in the universe to overcome the current rate of expansion. With the added repulsive force of dark energy, the expansion rate will increase, instead of decrease. Objects such as stars, galaxies, and clusters, which are kept together by their own self-gravity, will remain largely intact, but the enormous voids separating clusters and superclusters from each other will grow ever larger. Eventually, over time scales of trillions of years, the universe will grow empty and dim, illuminated by only a few last stellar remnants, cooling slowly toward absolute zero.
Conclusion
Over the past several centuries, astronomers have assembled a fairly comprehensive and consistent view of the universe. Many of the major constituents are now known, while modern astronomical theories offer plausible explanations of how they were formed, what they are made of, and how they interact with each other. There are, however, a number of key unanswered questions, for example, the nature of dark matter and dark energy, and whether the four fundamental forces merge into a grand unified force at extremely high temperatures and pressures consistent with the conditions of the early universe. These questions will require many further advances in technology, observation, and theory to resolve fully. See also: Cosmology